The team at the Nanomaterials, Mechanics, and MEMS Laboratory is focused on the synthesis and characterization of...
There are a number of options and technologies available for digital imaging in transmission electron microscopy (TEM) applications today. Traditionally, high energy electrons could not be directly exposed to a sensor without excessively damaging the detector. As a consequence, conventional TEM cameras first expose the incoming electron beam to a scintillating film that converts the electrons into light (photons). These photons are then transferred to the sensor, either through a series of optical lenses or a coupled fiber optic plate. Finally, the light is collected by a sensor where the image is created pixel-by-pixel based on the amount of light detected at each position in the sensor.
Conventional TEM image detection architecture
There are four basic steps in TEM imaging to address incoming electrons:
- Convert electrons to signal
- Transfer signal
- Detect signal with sensor
- Electronically transfer signal and read-out to form image
What is different with direct detection?
There are only two steps in TEM imaging with direct detection:
- Convert electrons to signal – not applicable
- Transfer signal – not applicable
- Detect signal with sensor
- Electronically transfer signal and read-out to form image
One key difference between conventional and direct detection is a custom CMOS sensor that utilizes the only radiation hard architecture that can tolerate direct exposure to high energy particles. To add, extremely high speed electronics for data transfer and processing enable low-dose counting and super-resolution capabilities. Combined, this allows frame rates (4k x 4k) of 400 frames per second (fps) to be processed in real-time to achieve optimal results.
Convert electrons to signal Transfer signal Detect signal with sensor Transfer signal and read-out image
Step 1) Convert electrons to signal
Gatan uses proprietary phosphor scintillators to optimize signal conversion that enhances detector sensitivity (SENS) and resolution. When you select a scintillator, it is appropriate to know the performance trade-offs between SENS and resolution.
- Sensitivity (signal): Ideal for dose-sensitive use cases where you need to generate more photons per incoming electron (e.g., cryo-tomography, beam sensitive materials)
- Resolution (spatial detail): Favorable for applications where you require more information to resolve details, but you can increase the dose (signal) without harming the sample (e.g., semiconductors and other less-sensitive materials)
Step 2) Transfer signal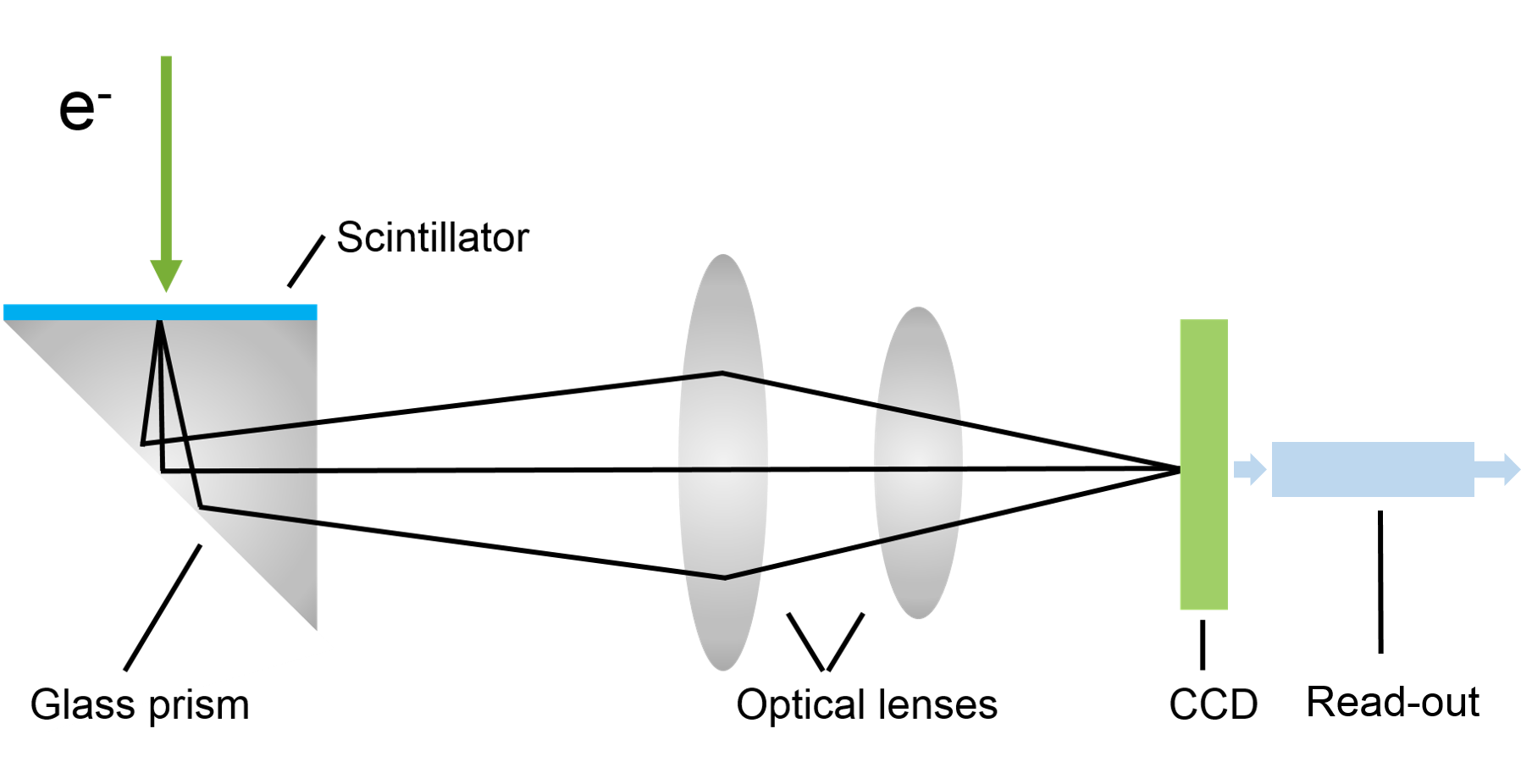
Various coupling (lens- and fiber-coupled) mechanisms are available to optimize signal transfer and meet cost or performance targets for a given detector.
Lens-coupled: Lens optics transmit light to the sensor where it is converted into sensor electrons (signal)
- Pros: (Gatan) Uses real transmission scintillator; can be less expensive than higher performance fibers
- Cons: Light (information) loss with lensing, angular dependencies (<10% efficient); vignetting (light fall-off); image distortion for higher magnification
Fiber-coupled: Scintillator creates photons that subsequently create sensor electrons; fiber directly transmits light to sensor with high efficiency
- Pros: Most efficient transmission of light information to the sensor (1:1 coupling of scintillator:sensor (>50% efficient) with no image distortion); can trade-off SENS verses resolution (fiber configuration detail)
- Cons: Fiber optics are slightly higher cost; requires process optimization including cladding, sintering, bonding of fibers (Gatan proprietary)
Step 3) Detect signal with sensor
Sensor type (CCD vs. CMOS) offers significant trade-offs for TEM camera performance as there are fundamental differences in architecture.
- Charge-coupled device (CCD): Charge transfers between neighboring cells, and read-out (e.g., noise) is seen at final stage; binning minimizes the impact of read-out noise
- Complementary metal–oxide–semiconductor (CMOS): Charge immediately converts to voltage (read-out with digital output); supports high frame rates, low overall electronics noise
Both technologies possess inherent advantages, so the question arises about what unique performance characteristics arise from each choice. CCDs can have 100% fill factor that captures all incoming light, whereas part of the CMOS sensor is occupied by transistors and metal wiring associated with each pixel. Historically, CCDs provided higher quality images with low noise, at affordable prices. Recent design advancements and processing techniques now advance CMOS sensor performance so it is a viable choice for some applications. Note that CCDs still maintain an advantage for binning in terms of signal-to-noise. However, CMOS chips can scale the number of read-out ports and achieve very high frame rates.
Step 4) Transfer signal and read-out image
When a charge converts to voltage, you typically generate noise
- CCD: Transfer data out of serial register
- CMOS: Converts to voltage per pixel
It is very important to optimize read-out noise (higher voltages) and speed (multi-port and fast read times) for CCDs.
- Optimize controller for low read-noise; leverage multi-port read-outs for faster speed
- Interline CCDs with binning have the fastest readouts (fps) – up to 30 fps due to 100% duty cycle
CMOS typically is seen as a fast sensor because you can run in rolling shutter mode verses the slow global shutter mode.
The cutting-edge counting camera for groundbreaking imaging, diffraction, and in-situ studies.
The only fully integrated hybrid-pixel electron detector with the Gatan Microscopy Suite software for advanced electron diffraction studies.
Gatan’s latest CMOS camera that with its resolution, speed, and ease of use will revolutionize electron microscopy.
Facilitate your HREM assays by automatically adjusting the critical imaging parameters of a TEM microscope focus, stigmation, and beam tilt.
DigitalMicrograph, also known as Gatan Microscopy Suite, drives your digital cameras and surrounding components to support key applications including tomography, in-situ, spectrum and diffraction imaging, plus more.
Delivers the efficiency and high-throughput data collection that you expect from Latitude software to MicroED studies.
Digital beam control and image processing to enhance the photographic quality of your digital images.
Diffraction analysis package (DIFPACK) to automate the selection area of your electron diffraction (SAED) patterns and high resolution lattice images of crystalline samples.
A powerful tool that adds 4D STEM diffraction capabilities to your existing Gatan in-situ camera.
Sets a new standard for the efficient, high-throughput collection of low-dose, single-particle, cryo-EM datasets from Gatan’s cameras.
The high-performance scintillator camera that elevates your everyday transmission electron microscopy
eBIC for Industry is a recently inaugurated center offering professional cryo-EM services to the global pharmaceutical and biotech industry.
-
GIF Continuum K3 IS: Advanced Direct Detection for In-Situ Chemical Analysis
-
Capturing, Processing, and Synchronizing In-Situ EELS Data
-
Continuum IS: Versatile time-resolved data collection webinar
-
Continuously acquired 4D STEM and EELS spectrum images for in-situ microscopy webinar
-
4D STEM and Virtual Aperture Imaging with ClearView
-
Image samples at the desired orientation with TruAlign
-
Better resolve faint, high-resolution diffraction spots with ClearView Frame Control mode
-
High signal-to-noise TEM imaging with ClearView Frame Control mode
-
Live drift correction during imaging acquisition
-
High-resolution imaging of lithium-ion battery materials
In situ structural analysis of SARS-CoV-2 spike reveals flexibility mediated by three hinges
Turoňová, B.; Sikora, M.; Schürmann, C.; Hagen, W. J. H.; Welsch, S.; Blanc, F. E. C.; von Bülow, S.; Gecht, M.; Bagola, K.; Hörner, C.; van Zandbergen, G.; Landry, J.; de Azevedo, N. T. D.; Mosalaganti, S.; Schwarz, A.; Covino, R.; Mühlebach, M. D.; Hummer, G.; Locker, J. K.; Beck, M.
Molecular architecture of the SARS-CoV-2 virus
Yao, H.; Song, Y.; Chen, Y.; Wu, N.; Xu, Sun, C.; Zhang, J.; Weng, T.; Zhang, Z.; Wu, Z.; Cheng, L,; Shi, D.; Lu, X.; Lei, J.; Crispin, M.; Shi, Y.; Li, L.; Li, S.
Atomic-resolution transmission electron microscopy of electron beam–sensitive crystalline materials
Zhang, D.; Zhu, Y.; Liu, L.; Ying, X.; Hsiung, C. -E.; Sougrat, R.; Li, K.; Han, Y.
3D structure of individual nanocrystals in solution by electron microscopy
Park, J.; Elmlund, H.; Ercius, P.; Yuk, J. M.; Limmer, D. T.; Chen, Q.; Kim, K.; Han, S. H.; Weitz, D. A.; Zettl, A.; Paul Alivisatos, A. P.
2.2 Å resolution cryo-EM structure of β-galactosidase in complex with a cell-permeant inhibitor
Bartesaghi, A.; Merk, A.; Banerjee, S.; Matthies, D.; Wu, X.; Milne, J. L. S.; Subramaniam, S.
Atomic structure of anthrax protective antigen pore elucidates toxin translocation
Jiang, J.; Pentelute, B. L.; Collier, R. J.; Zhou, Z. H.
Campbell, M. G.; Veesler, D.; Cheng, A.; Potter, C. S.; Carragher, B.
Three-dimensional structure of human γ-secretase
Lu, P.; Bai, X.; Ma, D.; Xie, T.; Yan, C.; Sun, L.; Yang, G.; Zhao, Y.; Zhou, R.; Scheres, S. H.; Shi, Y.
Structure of the TRPV1 ion channel determined by electron cryo-microscopy
Liao, M.; Cao, E.; Julius, D.; Cheng, Y.
Li, X.; Mooney, P.; Zheng, S.; Booth, C. R.; Braunfeld, M. B.; Gubbens, S.; Agard, D. A.; Cheng, Y.
Multi-convergence-angle ptychography with simultaneous strong contrast and high resolution
Mao, W.; Zhang, W.; Huang, C.; Zhou, L.; Kim, J. S.; Gao, S.; Lei, Y.; Wu, X.; Hu, Y.; Pei, X.; Fang, W.; Liu, X.; Song, J.; Fan, C.; Nie, Y.; Kirkland, A. I.; Wang, P.
Chen, Y.; Chou, T. -C.; Fang, C. -H.; Lu, C. -Y.; Hsiao, C. -N.; Hsu, W. -T.; Chen, C. -C.
Reassessing chain tilt in the lamellar crystals of polyethylene
Kanomi, S.; Marubayashi, H.; Miyata, T.; Jinnai, H.
Hogan-Lamarre, P.; Luo, Y.; Bücker, R.; Miller, R. J. D.; Zou, X.
Electron-counting MicroED data with the K2 and K3 direct electron detectors
Clabbers, M. T. B.; Martynawycz, M. W.; Hattne, J.; Nannenga, B. L.; Gonen, T.
Yang, T.; Xua, H.; Zoua, X.
Intermetallic nanocrystal discovery through modulation of atom stacking hierarchy
Du, J. S.; Dravid, V. P.; Mirkin, C. A.
Hölzel, H.; Lee, S.; Amsharov, K.; Jux, N.; Harano, K.; Nakamura, E.; Lungerich, D.
Kuwahara, M.; Mizuno, L.; Yokoi, R.; Morishita, H.; Ishida, T.; Saitoh, K.; Tanaka, N.; Kuwahara, S.; Agemura, T.
Organic crystal growth: Hierarchical self-assembly involving nonclassical and classical steps
Biran, I.; Rosenne, S.; Weissman, H.; Tsarfati, Y.; Houben, L.; Rybtchinski. B.
Vanacore, G. M.;Chrastina, D.; Scalise, E.; Barbisan, L.; Ballabio, A.; Mauceri, M.; Via, F. L.; Capitani, G.; Crippa, D.; Marzegalli, A.; Bergamaschini. R.; Miglio. L.
Deagglomeration of DNA nanomedicine carriers using controlled ultrasonication
Hinchliffe, B. A.; Turner, P.; J. H. Cant, D.; De Santis, E.; Aggarwal, P.; Harris, R.; Templeton, D.; Shard, A. G.; Hodnett, M.; Minelli, C.
Three-dimensional electron ptychography of organic–inorganic hybrid nanostructures
Ding, Z.; Gao, S.; Fang, W.; Huang, C.; Zhou, L.; Pei, X.; Liu, X.; Pan, X.; Fan, C.; Kirkland, A. I.; Wang, P.
The performance of detectors for diffraction-based studies in (S)TEM
Pakzad, A.; dos Reis. R. D.
How to get something out of nothing (almost!): Extracting information from noisy data
Crozier, P. A.
Orris, B.; Huynh, K. W.; Ammirati, M.; Han, S.; Bolaños, B.; Carmody, J.; Petroski, M. D.; Bosbach, B.; Shields, D. J.; Stivers, J. T.
Structural analysis of the basal state of the Artemis:DNA-PKcs complex
Watanabe, G.; Lieber, M. R.; Williams, D. R.
Shimizu, T.; Lungerich, D.; Harano, K.; Nakamura, E.
Hasegawa, S.; Masuda, S.; Takano, S.; Harano, K.; Tsukuda, T.
Liu, D.; Kowashi, S.; Nakamuro, T.; Lungerich, D.; Yamanouchi, K.; Harano, K.; Nakamura, E.
Metastable hexagonal close-packed palladium hydride in liquid cell TEM
Hong, J.; Bae, J. -H.; Jo, H.; Park, H. -Y.; Lee, S.; Hong, S. J.; Chun, H.; Cho, M. K.; Kim, J.; Kim, J.; Son, Y.; Jin, H.; Suh, J. -Y.; Kim, S. -C.; Roh, H. -K.; Lee, K. H.; Kim, H. -S.; Chung, K. Y.; Yoon, C. W.; Lee, K.; Kim, S. H.; Ahn, J. -P.; Baik, H.; Kim, G. H.; Han, B.; Jin, S.; Hyeon, T.; Park, J.; Son, C. Y.; Yang, Y.; Lee, Y. -S.; Yoo, S. J.; Chun, D. W.
Potential nanoscale sources of decoherence in niobium based transmon Qubit architectures
Murthy, A. A.; Das, P. M.; Ribet, S. M.; Kopas, C.; Lee, J.; Reagor, M. J.; Zhou, L.; Kramer, M. J.; Hersam, M. C.; Checchin, M.; Grassellino, A.; dos Reis, R.; Dravid, V. P.; Romanenko, A.
High-density switchable skyrmion-like polar nanodomains integrated on silicon
Han, L.; Addiego, C.; Prokhorenko, S.; Wang, M.; Fu, H.; Nahas, Y.; Yan, X.; Cai, S.; Wei, T.; Fang, Y.; Liu, H.; Ji, D.; Guo, W.; Gu, Z.; Yang, Y.; Wang, P.; Bellaiche, L.; Chen, Y.; Wu, D.; Nie, Y.; Pan, X.
Imaging of isotope diffusion using atomic-scale vibrational spectroscopy
Senga, R.; Lin, Y. -C.; Morishita, S.; Kato, R.; Yamada, T.; Hasegawa, M.; Suenaga, K.
Liu, D.; Kowashi, S.; Nakamuro, T.; Lungerich, D.; Yamanouchi, K.; Harano, K.; Nakamura, E.
Kim, S. C.; Huang, W.; Zhang, Z.; Wang, J.; Kim, Y.; Jeong, Y. K.; Oyakhire, S. T.; Yang, Y.; Cui, Y.
The giant Mimivirus 1.2 Mb genome is elegantly organized into a 30 nm helical protein shield
Villalta, A.; Schmitt, A.; Estrozi, L. F.; Quemin, E. R. J.; Alempic, J. -M.; Lartigue, A.; Pražák, V.; Belmudes, L.; Vasishtan, D.; Colmant, A. M. G.; Honoré, F. A.; Couté, Y.; Grünewald, K.; Abergel, C.
Donohue, J.; Zeltmann, S. E.; Bustillo, K. C.; Savitzky, B.; Jones, M. A.; Meyers, G.; Ophus, C.; Minor, A. M.
Lattice-resolution, dynamic imaging of hydrogen absorption into bimetallic AgPd nanoparticles
Angell, D. K.; Bourgeois, B.; Vadai, M.; Dionne, J. A.
Suspension electrolyte with modified Li+ solvation environment for lithium metal batteries
Kim, M. S.; Zhang, Z.; Rudnicki, P. E.; Yu, Z.; Wang, J.; Wang, H.; Oyakhire, S. T.; Chen, Y.; Kim, S. C.; Zhang, W.; Boyle, D. T.; Kong, X.; Xu, R.; Huang, Z.; Huang, W.; Bent, S. F.; Wang, L. -W.; Qin, J.; Bao, Z.; Cui , Y.
Rational solvent molecule tuning for high-performance lithium metal battery electrolytes
Yu, Z.; Rudnicki, P. E.; Zhang, Z.; Huang, Z.; Celik, H.; Oyakhire, S. T.; Chen, Y.; Kong, X.; Kim, S. C.; Xiao, X.; Wang, H.; Zheng, Y.; Kamat, G. A.; Kim, M. S.; Bent, S. F.; Qin, J.; Cui, Y.; Bao, Z.
Capturing the swelling of solid-electrolyte interphase in lithium metal batteries
Zhang, Z.; Li, Y.; Xu, R.; Zhou, W.; Li, Y.; Oyakhire, S. T.; Wu, Y.; Xu, J.; Wang, H.; Yu, Z.; Boyle, D. T.; Huang, W.; Ye, Y.; Chen, H.; Wan, J.; Bao, Z.; Chiu, W.; Cui, Y.
Electric field control of chirality
Behera, P.; May, M. A.; Gomez-Ortiz, F.; Susarla, S.; Das, S.; Nelson, C. T.; Caretta, L.; Hsu, S. -L.; McCarter, M. R.; Savitzky, B. H.; Barnard, E. S.; Raja, A.; Hong, Z.; Garcia-Fernandez, P.; Lovesey, S. W.; Van der Laan, G.; Ercius, P.; Ophus, C.; Martin, L. W.; Junquera, J.; Raschke, M. B.; Ramesh, R.
Spinterface induced modification in magnetic properties in Co40F e40B20/fullerene bilayers
Sharangi, P.; Pandey, E.; Mohanty,S.; Nayak, S.; Bedanta, S.
Automated crystal orientation mapping in py4DSTEM using sparse correlation matching
Ophus, C.; Zeltmann, S. E.; Bruefach, A.; Rakowski, A.; Savitzky, B. H.; Minor, A. M.; Scott, M. C.
De novo synthesis of free-standing flexible 2-D intercalated nanofilm uniform over tens of cm2
Ravat, P.; Uchida, H.; Sekine, R.; Kamei, K.; Yamamoto, A.; Konovalov, O.; Tanaka, M.; Yamada, T.; Harano, K.; Nakamura, E.
Spatial mapping of electrostatic fields in 2D heterostructures
Murthy, A. A.; Ribet, S. M.; Stanev, T. K.; Liu, P.; Watanabe, K.; Taniguchi, T.; Stern, N. P.; dos Reis, R.; Dravid, V. P.
A simple pressure-assisted method for MicroED specimen preparation
Zhao, J.; Xu, H.; Lebrette, H.; Carroni, M.; Taberman, H.; Hogbom, M.; Zou, X.
Electron crystallographic investigation of crystals on the mesostructural scale
Mao, W.; Bao, C.; Han, L.
Atomic-number (Z)-correlated atomic sizes for deciphering electron microscopic molecular images
Xing, J.; Takeuchi, K.; Kamei, K.; Nakamuro, T.; Harano, K.; Nakamura, E.
Nanodiffraction imaging of polymer crystals
Kanomi, S.; Marubayashi, H.; Miyata, T.; Tsuda, K.; Jinnai, H.
Nikishin, I.; Dulimov, R.; Skryabin, G.; Galetsky, S.; Tchevkina, E.; Bagrov, D.
Lungerich, D.; Hoelzel, H.; Harano, K.; Jux, N.; Amsharov, K. Y.; Nakamura, E.
Lungerich, D.; Hoelzel, H.; Harano, K.; Jux, N.; Amsharov, K. Y.; Nakamura, E.
Intrinsic helical twist and chirality in ultrathin tellurium nanowires
Londoño-Calderon, A.; Williams, D. J.; Schneider, M. M.; Savitzky, B. H.; Ophus, C.; Ma, S.; Zhud, H.; Pettes, M. T.
Direct visualization of the earliest stages of crystallization
Singh, M. K.; Ghosh, C.; Miller, B.; Carter, C. B.
Dual-solvent Li-ion solvation enables high-performance Li-metal batteries
Wang, H.; Yu, Z.; Kong, X.; Huang, W.; Zhang, Z.; Mackanic, D. G.; Huang, X.; Qin, J.; Bao, Z.; Cui. Y.
Hasegawa, S.; Takano, S.; Harano, K.; Tsukuda, T.
Hasegawa, S.; Takano, S.; Harano, K.; Tsukuda, T.
Rim binding of cyclodextrins in size-sensitive guest recognition
Hanayama, H.; Yamada, J.; Tomotsuka, I.; Harano, K.; Nakamura, E.
Graded microstructure preparation in austenitic stainless steel during radial-shear rolling
Lezhnev, S. N.; Naizabekov, A. B.; Panin, E. A.; Volokitina, I. E.; Arbuz, A. S.
Corrosion of lithium metal anodes during calendar ageing and its microscopic origins
Boyle, D. T.; Huang, W.; Wang, H.; Li, Y.; Chen, H.; Yu, Z.; Zhang, W.; Bao, Z.; Cui, Y.
Few-nm-sized, phase-pure Au5Sn intermetallic nanoparticles: synthesis and characterization
Osugi, S.; Takano, S.; Masuda, S.; Harano, K.; Tsukuda, T.
Cheng, Q.; Li, Q.; Xu, L.; Jiang, H.
Darling, A. L.; Dahrendorff, J.; Creodore, S. G.; Dickey, C. A.; Blair, L. J.; Uversky, V. N.
Evolution of anisotropic arrow nanostructures during controlled overgrowth
Wang, W.; Erofeev, I.; Nandi, P.; Yan, H.; Mirsaidov, U.
Local lattice deformation of tellurene grain boundaries by four-dimensional electron microscopy
Londoño-Calderon, A.; J. Williams, D. J.; Schneider, M.; Savitzky, B. H.; Ophus, C.; Pettes, M. T.
Mapping grains, boundaries, and defects in 2D covalent organic framework thin films
Castano, I.; Evans, A. M.; dos Reis, R,; Dravid, V. P.; Gianneschi, N. C.; Dichtel, W. R.
Au/TiN nanostructure materials for energy storage applications
Ali, S. M.; Ramay, S. M.; Mahmood, A.; ur Rehman, A.; Ali, G.; Ali, S. D.; Uzzaman, T.
Sekine, R.; Ravat, P.; Yanagisawa, H.; Liu, C.; Kikkawa, M.; Harano, K.; Nakamura, E.
SINGLE: Atomic-resolution structure identification of nanocrystals by graphene liquid cell EM
Reboul, C. F.; Heo, J.; Machello, C.; Kiesewetter, S.; Kim, B. H.; Kim, S.; Elmlund, D.; Ercius, P.; Park, J.; Elmlund, H.
Hu, S.; Yin, Y.; Chen, B.; Lin, Q.; Tian, Y.; Song, X.; Peng, J.; Zheng, H.; Rao, S.; Wu, G.; Mo, X.; Yan, F.; Chen, J.; Lu, Y.
Subramanian, V.; Rodemoyer, B.; Shastri, V.; Rasmussen, L. J.; Desler, C.; Schmidt, K. H.
Capturing the moment of emergence of crystal nucleus from disorder
Nakamuro, T.; Sakakibara, M.; Nada, H.; Harano, K.;Nakamura, E.
4D imaging of soft matter in liquid water
Marchello, G.; De Pace, C.; Acosta-Gutierrez, S.; Lopez-Vazquez, C.; Wilkinson, N.; Gervasio, F. L.; Ruiz-Perez, L.; Giuseppe Battaglia, G.
Greaney, A. J.; Starr, T. N.; Gilchuk, P.; Zost, S. J.; Binshtein, E.; Loes, A. N.; Hilton, S. K.; Huddleston, J.; Eguia, R.; Crawford, K. H. D.; Dingens, A. S.; Nargi, R. S.; Sutton, R. E.; Suryadevara, N.; Rothlauf, P. W.; Liu, Z.; Whelan, S. P. J.; Carnahan, R. H.; Bloom, J. D.
Mechanism of SARS-CoV-2 polymerase stalling by remdesivir
Kokic, G.; Hillen, H. S.; Tegunov, D.; Dienemann, C.; Seitz, F.; Schmitzova, J.; Farnung, L.; Siewert, A.; Höbartner, C.; Cramer, P.
Development and structural basis of a two-MAb cocktail for treating SARS-CoV-2 infections
Zhang, C.; Wang, Y.; Zhu, Y.; Liu, C.; Gu, C.; Xu, S.; Wang, Y.; Zhou, Y.; Wang, Y.; Han, W.; Hong, X.; Yang, Y.; Zhang, X.; Wang, T.; Xu, C.; Hong, Q.; Wang, S.; Zhao, Q.; Qiao, W.; Zang, J.; Kong, L.; Wang, F.; Wang, H.; Qu, D.; Lavillette, D.; Tang, H.; Deng, Q.; Xie, Y.; Cong, Y.; Huang, Z.
Stabilizing the closed SARS-CoV-2 spike trimer
Juraszek, J.; Rutten, L.; Blokland, S.; Bouchier, P.; Voorzaat, R.; Ritschel, T.; Bakkers, M. J. G.; Renault , L. L. R.; Langedijk, J. P. M.
Yan, L.; Ge, J.; Zheng, L.; Zhang, Y.; Gao, Y.; Wang, T.; Huang, Y.; Yang, Y.; Gao, S.; Li, M.; Liu, Z.; Wang, H.; Li, Y.; Chen, Y.; Guddat, L. W.; Wang, Q.; Rao, Z.; Lou, Z.
Xu, C.; Wang, Y.; Liu, C.; Zhang, C.; Han, W.; Hong, X.; Wang, Y.; Hong, Q.; Wang, S.; Zhao, Q.; Wang, Y.; Yang, Y.; Chen, K.; Zheng, W.; Kong, L.; Wang, F.; Zuo, Q.; Huang, Z.; Cong, Y.
Spatial mapping of electrostatics and dynamics across 2D heterostructures
Murthy, A. A.; Stanev, T. K.; Ribet, S. M.; Liu, P.; Watanabe, K.; Taniguchi, T.; Stern, N. P.; dos Reis, R.; Dravid, V. P.
Mihelc. E. M.; Baker, S. C.; Lanman, J. K.
Yuan, S.; Peng, L.; Park, J. J.; Hu, Y.; Devarkar, S. C.; Dong, M. B.; Shen, Q.; Wu, S.; Chen, S.; Lomakin, I. B.; Xiong, Y.
Zhou, T.; Tsybovsky, Y.; Gorman, J.; Rapp, M.; Cerutti, G.; Chuang, G. -Y.; Katsamba, P. S.; Sampson, J. M.; Schön, A.; Bimela, J.; Boyington, J. C.; Nazzari, A.; Olia, A. S.; Shi, W.; Sastry, M.; Stephens, T.; Stuckey, J.; Teng, I. -T.; Kwong, P. D
Electronically coupled 2D polymer/MoS2 heterostructures
Balch, H. B., Evans, A. M., Dasari, R. R., Li, H., Li, R., Thomas, S., Wang, Q., Bisbey, R. P., Slicker, K., Castano, I., Xun, S., Jiang, L., Zhu, C., Gianneschi, N., Ralph, D. C., Brédas, J-L., Marder, S. R., Dichtel, W. R., Wang, F.
De novo design of potent and resilient hACE2 decoys to neutralize SARS-CoV-2
Linsky, T. W.; Vergara, R.; Codina, N.; Nelson, J. W.; Walker, M. J.; Su, W.; Barnes, C. O.; Hsiang, T. -Y.; Esser-Nobis, K.; Yu, K.; Reneer, B.; Hou, Y. J.; Priya, T.; Mitsumoto, M.; Pong, A.; Lau, Y.; Mason, M. L.; Chen, J.; Chen, A.; Berrocal, T.; Peng, H.; Clairmont, N. S.; Castellanos, J.; Lin, Y. -R..; Josephson-Day, A.; Baric, R. S.; Fuller, D. H.; Walkey, C. D.; Ross, T. M.; Swanson, R.; Bjorkman, P. J.; Gale Jr., M.; Blancas-Mejia, L. M.; Yen, H. -L.; Silva, D. -A.
Yao, H.; Sun, Y.; Deng, Y. -Q.; Wang, N.; Tan, Y.; Zhang, N. -N.; Li, X. -F.; Kong, C.; Xu, Y. -P.; Chen, Q.; Cao, T. -S.; Zhao, H.; Yan, X.; Cao, L.; Lv, Z.; Zhu, D.; Feng, R.; Wu, N.; Zhang, W.; Hu, Y.; Chen, K.; Zhang, R. -R.; Lv, Q.; Sun, S.; Zhou, Y.; Yan, R.; Yang, G.; Sun, X.; Liu, C.; Lu, X.; Cheng, L.; Qiu, H.; Huang, X. -Y.; Weng, T.; Shi, D.; Jiang, W.; Shao, J.; Wang, L.; Zhang, J.; Jiang, T.; Lang, G.; Qin, C. -F.; Li, L.; Wang, X.
Structure-based development of human antibody cocktails against SARS-CoV-2
Wang, N.; Sun, Y.; Feng, R.; Wang, Y.; Guo, Y.; Zhang, L.; Deng, Y. -Q.; Wang, L.; Cui, Z.; Cao, L.; Zhang, Y. -J.; Li, W.; Zhu, F. -C.; Qin, C. -F.; Wang, X.
Structural analysis of full-length SARS-CoV-2 spike protein from an advanced vaccine candidate
Bangaru, S.; Ozorowski, G.; Turner, H. L.; Antanasijevic, A.; Huang, D.; Wang, X.; Torres, J. L.; Diedrich, J. K.; Tian, J. -H.; Portnoff, A. D.; Patel, N.; Massare, M. J.; Yates III, J. R.; Nemazee, D.; Paulson, J. C.; Glenn, G.; Smith, G.; Ward, A. B.
Ultrapotent human antibodies protect against SARS-CoV-2 challenge via multiple mechanisms
Tortorici, M. A.; Beltramello, M.; Lempp, F. A.; Pinto, D.; Dang, H. V.; Rosen, L. E.; McCallum, M.; Bowen, J.; Minola, A.; Jaconi, S.; Zatta, F.; De Marco, A.; Guarino, B.; Bianchi, S.; Lauron, E. J.; Tucker, H.; Zhou, J.; Peter, A.; Havenar-Daughton, C.; Wojcechowskyj, J. A.; Case, J. B.; Chen, R. E.; Kaiser, H.; Montiel-Ruiz, M.; Meury, M.; Czudnochowski, N.; Spreafico, R.; Dillen, J.; Ng, C.; Sprugasci, N.; Culap, K.; Benigni, F.; Abdelnabi, R.; Foo, S. -Y. C.; Schmid, M. A.; Cameroni, E.; Riva, A.; Gabrieli, A.; Galli, M.; Pizzuto. M. S.; Neyts, J.; Diamond, M. S.; Virgin, H. W.; Snell, G.; Corti, D.; Fink, K.; Veesler, D.;
Architecture of a SARS-CoV-2 mini replication and transcription complex
Yan, L.; Zhang, Y.; Ge, J.; Zheng, L.; Gao, Y.; Wang, T.; Jia, Z.; Wang, H.; Huang, Y.; Li, M.; Wang, Q.; Ra, Z.; Lou, Z.
Du, S.; Cao, Y.; Zhu, Q.; Yu, P.; Qi, F.; Wang, G.; Du, X.; Bao, L.; Deng, W.; Zhu, H.; Liu, J.; Nie, J.; Zheng, Y.; Liang, H.; Liu, R.; Gong, S.; Xu, H.; Yisimayi, A.; Qin, C.
Piccoli, L.; Park, Y. -J.; Tortorici, M. A.; Czudnochowski, N.; Walls, A. C.; Beltramello, M.; Silacci-Fregni, C.; Pinto, D.; Rosen, L. E.; Bowen, J. E.; Acton, O. J.; Jaconi, S.; Guarino, B.; Minola, A.; Zatta, F.; Sprugasci, N.; Bassi, J.; Peter, A.; De Marco, A.; Nix, J. C.; Mele, F.; Jovic, S.; Rodriguez, B. F.; Gupta, S. V.; Jin, F.; Piumatti, G.; Presti, G. L.; Pellanda, A. F.; Biggiogero, M.; Tarkowski, M.; Pizzuto, M. S.; Cameroni, E.; Havenar-Daughton, C.; Smithey, M.; Hong, D.; Lepori, V.; Albanese, E.; Ceschi, A.; Bernasconi, E.; Elzi, L.; Ferrari, P.; Garzoni, C.; Riva, A.; Snell, G.; Sallusto, F.; Fink, K.; Virgin, H. W.; Lanzavecchia, A.; Corti, D.; Veesler, D.
Guo, L.; Bi, W.; Wang, X.; Xu, W.; Yan, R.; Zhang, Y.; Zhao, K.; Li, Y.; Zhang, M.; Cai, X.; Jiang, S.; Xie, Y.; Zhou, Q.; Lu, L.; Dang, B.
Cathode-electrolyte interphase in lithium batteries revealed by cryogenic electron microscopy
Zhang, Z.; Yang, J.; Huang, W.; Wang, H.; Zhou, W.; Li, Y.; Li, Y.; Xu, J.; Huang, W.; Chiu, W.
Free fatty acid binding pocket in the locked structure of SARS-CoV-2 spike protein
Toelzer, C.; Gupta, K.; Yadav, S. K. N.: Borucu, U.; Davidson, A. D.; Williamson, M. K.; Shoemark, D. K.; Garzoni, F.; Staufer, O.; Milligan, R.; Capin, J.; Mulholland, A. J.; Spatz, J.; Fitzgerald, D.; Berger, I.; Schaffitzel, C.
An ultrapotent synthetic nanobody neutralizes SARS-CoV-2 by stabilizing inactive spike
Schoof, M.; Faust, B.; Saunders, R. A.; Sangwan, S.; Rezelj, V.; Hoppe, N.; Boone, M.; Billesbølle, C. B.; Puchades, C.; Azumaya, C. M.; Kratochvil, H. T.; Zimanyi, M.; Deshpande, I.; Liang, J.; Dickinson, S.; Nguyen, H. C.; Chio, C. M.; Merz, G. E.; Thompson, M. C.; Diwanji, D.; Schaefer, K.; Anand, A. A.; Dobzinski, N.; Zha, B. S.; Simoneau, C. R.; Leon, K.; White, K. M.; Chio, U. S.; Gupta, M.; Jin, M.; Li, F.; Liu, Y.; Zhang, K.; Bulkley, D.; Sun, M.; Smith, A. M.; Rizo, A. N.; Moss, F.; Brilot, A. F.; Pourmal, S.; Trenker, R.; Pospiech, T.; Gupta, S.; Barsi-Rhyne, B.; Belyy, V.; Barile-Hill, A. W.; Nock, S.; Liu, Y.; Krogan, N. J.; Ralston, C. Y.; Swaney, D. L.; García-Sastre, A.; Ott, M.; Vignuzzi, M.; QCRG Structural Biology Consortium; Walter, P.; Manglik, A.
Custódio, T. F.; Das, H.; Sheward, D. J.; Hanke, L.; Pazicky, S.; Pieprzyk, J.; Sorgenfrei, M.; Schroer, M. A.; Gruzinov, A. Y.; Jeffries, C. M.; Graewert, M. A.; Svergun, D. I.; Dobrev, N.; Remans, K.; Seeger, M. A.; McInerney, G. M.; Murrell, B.; Hällberg, B. M.; Löw, C.
The architecture of inactivated SARS-CoV-2 with postfusion spikes revealed by cryo-EM and cryo-ET
Liu, C.; Mendonça, L.; Yang, Y.; Gao, Y.; Shen, C.; Liu, J.; Ni, T.; Ju, B.; Liu, C.; Tang, X.; Wei, J.; Ma, X.; Zhu, Y.; Liu, W.; Xu, S.; Liu, Y.; Yuan, J.; Wu, J.; Liu, Z.; Zhang, Z.; Liu, L.; Wang, P.; Zhang, P.
Zhou, T.; Teng, I. -T.; Olia, A. S.; Cerutti, G.; Gorman, J.; Nazzari, A.; Shi, W.; Tsybovsky, Y.; Wang, L.; Wang, S.; Zhang, B.; Zhang, Y.; Katsamba, P. S.; Petrova, Y.; Banach, B. B.; Fahad, A. S.; Liu, L.; Lopez Acevedo, S. N.; Madan, B.; de Souza, M. O.; Pan, X.; Wang, P.; Wolfe, J. R.; Yin, M.; Ho, D. D.; Phung, E.; DiPiazza, A.; Chang, L. A.; Abiona, O. M.; Corbett, K. S.; DeKosky, B. J.; Graham, B. S.; Mascola, J. R.; Misasi, J.; Ruckwardt, T.; Sullivan, N. J.; Shapiro, L.; Kwong, P. D.
Kratish, Y.; Nakamuro, T.; Liu, Y.; Li, J.; Tomotsuka, I.; Harano, K.; Nakamura, E.; Marks, T. J.
InsteaDMatic: Towards cross-platform automated continuous rotation electron diffraction
Roslova, M.; Smeets, S.; Wang, B.; Thersleff, T.; Xu, H.; Zou, X.
In situ TEM study of crystallization and chemical changes in an oxidized uncapped Ge2Sb2Te5 film
Singh, M. K.; Ghosh, C.; Miller, B.; Kotula, P. G.; Tripathi, S.; Watt, J.; Bakan, G.; Silva, H.; Carter, C. B.
Inside polyMOFs: Layered structures in polymer-based metal–organic frameworks
Bentz, K. C., Gnanasekaran, K., Bailey, J. B. Ayala, S., Tezcan, F. A., Gianneschi, N. C., Cohen, S. M.
Aryl radical addition to curvatures of carbon nanohorns for single-molecule level molecular imaging
Kamei, K.; Shimizu, T.; Harano, K.; Nakamura, E.
Kim, Y. -J.; Lee, Y.; Kim, K.; Kwon, O. -H.
Effect of adventitious carbon on pit formation of monolayer MoS2
Park, S.; Siahrostami, S.; Park, J.; Mostaghimi, A. H. B.; Kim, T. R.; Vallez, L.; Gill, T. M.; Park, W.; Goodson, K. E.; Sinclair, R.; Zheng, X.
Stuckner, J.; Shimizu, T.; Harano, K.; Nakamura, E.; Murayama, M.
Gnanasekaran, K., Vailonis, K. M., Jenkins, D. M., Gianneschi, N. C.
Shimizu, T.; Lungerich, D.; Stuckner, J.; Murayama, M.; Harano, K.; Nakamura, E.
Langer, L. M.; Gat, Y.; Bonneau, F.; Conti, E.
Decoding the stoichiometric composition and organisation of bacterial metabolosomes
Yang, M.; Simpson, D. M.; Wenner, N.; Brownridge, P.; Harman, V. M.; Hinton, J. C. D.; Beynon, R. J.; Liu , L. -N.
1D to 2D transition in tellurium observed by 4D electron microscopy
Londoño-Calderon, A.; Williams, D. J.; Ophus, C.; Pettes, M. T.
Murthy, A. A.; Stanev, T. K.; dos Reis, R.; Hao, S.; Wolverton, C.; Stern, N. P.; Dravid, V. P.
Unveiling the microscopic origins of phase transformations: An in situ TEM perspective
Yu, L.; Hudak, B. M.; Ullah, A.; Thomas, M. P.; Porter, C. C.; Thisera, A.; Pham, R. H.; Goonatilleke, M. D. A.; Guiton, B. S.
Ogata, A. F.; Rakowski, A. M.; Carpenter, B. P.; Fishman, D. A.; Merham, J. G.; Hurst, P. J.; Patterson, J. P.
Thickness and defocus dependence of inter-atomic electric fields measured by scanning diffraction
Addiego, C.; Gao, W.; Pan, X.
Errokh, A.; Magnin, A.; Putaux, J. -L.; Boufi, S.
Nam, K. W.; Park, S. S.; dos Reis, R.; Dravid, V. P. Kim, H.; Mirkin, C. A.; Stoddart, J. F.
Gao, W.; Addiego, C.; Wang, H,; Yan, X.; Hou, Y.; Ji, D.; Heikes, C.; Zhang, Y.; Li, L.; Huyan, H.; Blum, T.; Aoki, T.; Nie, Y.; Schlom, D.; Wu, R.; Pan, X.
Buffin, S.; Peubez, I.; Barrière, F.; Nicolaï, M., -C.; Tapia, T.; Dhir, V.; Forma, E.; Sève, N.; Legastelois, I.
Recent progress of in situ transmission electron microscopy for energy materials
Chao Zhang, C.; Firestein, K. L.; Fernando, J. F. S.; Siriwardena, D.; von Treifeldt, J. E.; Golberg, D.
Electron microdiffraction reveals the nanoscale twist geometry of cellulose nanocrystals
Ogawa, Y.
Atomistic structures and dynamics of prenucleation clusters in MOF-2 and MOF-5 syntheses
Xing, J.; Schweighauser, L.; Okada, S.; Harano, K.; Nakamura, E.
Quantifying inactive lithium in lithium metal batteries
Fang, C.; Li, J.; Zhang, M.; Zhang, Y.; Yang, F.; Lee, J. Z.; Lee, L. M. -H.; Alvarado, J.; Schroeder, M. A.; Yang, Y.; Lu, B.; Williams, N.; Ceja, M.; Yang, L.; Cai, M.; Gu, J.; Xu, K.; Wang, X.; Meng, Y. S.
Improved applicability and robustness of fast cryo-electron tomography data acquisition
Eisenstein, F.; Danev, R.; Pilhofer, M.
Solving a new R2lox protein structure by microcrystal electron diffraction
Xu, H.; Lebrette, H.; Clabbers, M. T. B.; Zhao, J.; Griese, J. J.; Zou, X.; Hogbom, M.
Li, X.; Wang, J.; Liu, X.; Liu, L.; Cha, D.; Zheng, X.; Yousef, A. A.; Song, K.; Zhu, Y.; Zhang, D.; Han, Y.
Formation of two-dimensional transition metal oxide nanosheets with nanoparticles as intermediates
Yang, J.; Zeng, Z.; Kang, J.; Betzler, S.; Czarnik, C.; Zhang, X.; Ophus, C.; Yu, C.; Bustillo, K.; Pan, M.; Qiu, J.; Wang, L. -W.; Zheng, H.
Wang, H.; Liu, Z.; Liu, H.; Guan, L.; Cao, X.; Zhang, Z.; Huang, Y.; Jin, C.
Cryo-EM structures of atomic surfaces and host-guest chemistry in metal-organic frameworks
Li, Y.; Wang, K.; Zhou, W.; Sinclair, R.; Chiu, W.; Cui, Y.
Mechanism of stress induced crystallization of polyethylene
Yakovlev, S.; Fiscus, D.; Brant, P.; Butler, J.; Bucknall, D. G.; Downing, K. H.
Single particle cryo-EM reconstruction of 52 kDa streptavidin at 3.2 Angstrom resolution
Fan, X.; Wang, J.; Zhang, X.; Yang, Z.; Zhang, J. -C.; Zhao, L.; Peng, H. -L.; Lei, J.; Wang, H. -W.
Su, T.; Hood, Z. D.; Naguib, M.; Bai, L.; Luo, S.; Rouleau, C. M.; Ivanov, I. N.; Ji, H.; Qin, Z.; Wu, Z.
Thermal stability and irradiation response of nanocrystalline CoCrCuFeNi high-entropy alloy
Zhang, Y.; Tunes, M. A.; Crespillo, M. L.; Zhang, F.; Boldman, W. L.; Rack, P. D.; Jiang, L.; Xu, C.; Greaves, G.; Donnelly, S. E.; Wang, L.; Weber, W. J.
Tunes, M. A.; Harrison, R. W.; Donnelly, S. E.; Edmondson, P. D.
Factors affecting electron beam damage in calcite nanoparticles
Hooley, R.; Brown, A.; Brydson, R.
Low dose scanning transmission electron microscopy of organic crystals by scanning moiré fringes
S’ari, M.; Cattle, J.; Hondow, N.; Brydson, R.; Brown, A.
A 3.8 Å resolution cryo-EM structure of a small protein bound to an imaging scaffold
Liu, Y.; Huynh, D. T.; Yeates, T. O.
In situ structures of polar and lateral flagella revealed by cryo-electron tomography
Zhu, S.; Schniederberend, M.; Zhitnitsky, D.; Jain, R.; Galán, J. E.; Kazmierczak, B. I.; Liu, J.
Le Ferrand, H.; Duchamp, M.; Gabryelczyk, B.; Cai, H.; Miserez, A.
Carbon polyaniline capacitive deionization electrodes with stable cycle life
Evans, S. F.; Ivancevic, M. R.; Wilson, D. J.; Hood, Z. D.; Adhikari, S. P.; Naskar, A. K.; Tsouris, C.; Paranthaman, M. P.
Off-axis electron holography for imaging the magnetic behavior of vortex-state minerals
Almeida, T. P.; Muxworthy, A. R.; Williams, W.; Kasama, T.; Kovács, A.; Dunin-Borkowski, R. E.
Connolly, M.; Zhang, Y.; Mahri, S.; Brown, D. M.; Ortuño, N.; Jordá-Beneyto, M.; Maciaszek, K.; Stone, V.; Fernandes, T. F.; Johnston, H. J.
Mechanical response of gasoline soot nanoparticles under compression: An in situ TEM study
Jenei, I. Z.; Dassenoy, F.; Epicier, T.; Khajeh, A.; Martinic, A.; Uy, D.; Ghaednia, H.; Gangopadhyay, A.
2D/2D heterojunction of Ti3C2/g-C3N4 nanosheets for enhanced photocatalytic hydrogen evolution
Su, T.; Hood, Z. D.; Naguib, M.; Bai, L.; Luo, S.; Rouleau, C. M.; Ivanov, I. N.; Ji, H.; Qin, Z.; Wu, Z.
Rodriguez-Caro, H.; Dragovic, R.; Shen, M.; Dombi, E.; Mounce, G.; Field, K.; Meadows, J.; Turner, K.; Lunn, D.; Child, T.; Southcombe, J. H.; Granne, I.
Nanoscale mosaicity revealed in peptide microcrystals by scanning electron nanodiffraction
Gallagher-Jones, M.; Ophus, C.; Bustillo, K. C.; Boyer, D. R.; Panova, O.; Glynn, C.; Zee, C., -T.; Ciston, J.; Mancia, K. C.; Minor, A. M.; Rodriguez, J. A.
Unconventional magnetization textures and domain-wall pinning in Sm--Co magnets
Pierobon, L.; Kovács, A.; Schäublin, R. E.; Wyss, U.; Dunin-Borkowski, R. E.; Löffler, J. F.; Charilaou, M.
Structural analysis of single nanoparticles in liquid by low-dose STEM nanodiffraction
Khelfa, A.; Byun, C.; Nelayah, J.; Wang, G.; Ricolleau, C.; Alloyeau, D.
Surface crystallization of liquid Au–Si and its impact on catalysis
Panciera, F.; Tersoff, J.; Gamalski, A. D.; Reuter, M. C.; Zakharov, D.; Stach, E. A.; Hofmann, S.; Ross, F. M
Layered-structure SbPO4/reduced graphene oxide: An advanced anode material for sodium ion batteries
Pan, J.; Chen, S.; Fu, Q.; Sun, Y.; Zhang, Y.; Lin, N.; Gao, P.; Yang, J.; Qian, Y.
2D transition metal carbides (MXenes) for carbon capture
Persson, I.; Halim, J.; Lind, H.; Hansen, T. W.; Wagner, J. B.; Näslund, L. -A.; Darakchieva, V.; Palisaitis, J.; Rosen J.; Persson, P. O. A.
2D transition metal carbides (MXenes) for carbon capture
Persson, I.; Halim, J.; Lind, H.; Hansen, T. W.; Wagner, J. B.; Näslund, L. -A.; Darakchieva, V.; Palisaitis, J.; Rosen J.; Persson, P. O. A.
Gao, W.; Wu, J.; Yoon, A.; Lu, P.; Qi, L.; Wen, J.; Miller, D. J.; Mabon, J. C.; Wilson, W. L.; Yang, H.; Zuo, J. -M.
Atomic step flow on a nanofacet
Harmand, J. -C.; Patriarche, G.; Glas, F.; Panciera, F.; Florea, I.; Maurice, J. -L.; Travers, L.; Ollivier, Y.
A high-entropy alloy with hierarchical nanoprecipitates and ultrahigh strength
Fu, Z.; Jiang, L.; Wardini, J. L.; MacDonald, B. E.; Wen, H.; Xiong, W.; Zhang, D.; Zhou, Y.; Rupert, T. J.; Chen, W.; Lavernia, E. J.
Pan, J.; Chen, S.; Zhang, D.; Xu, X.; Sun, Y.; Tian, F.; Gao, P.; Yang, J.
Jung, H. J.; Kim, D.; Kim, S.; Park, J.; Dravid, V. P.; Shin, B.
In situ kinetic and thermodynamic growth control of Au–Pd core–shell nanoparticles
Tan, S. F.; Bisht, G.; Anand, U.; Bosman, M.; Yong, X. E.; Mirsaidov, U.
Yasin, F. S.; Harvey, T. R.; Chess, J. J.; Pierce, J. S.; Ophus, C.; Ercius, P.; McMorran, B. J.
Zeng, L.; Gammer, C.; Ozdol, B.; Nordqvist, T.; Nygård, J.; Krogstrup, P.; Minor, A. M.; Jäger, W.; Olsson, E.
Yu, J.; Yuan, W.; Yang, H.; Xu, Q.; Wang, Y.; Zhang, Z.
Refinement and analysis of the mature Zika virus cryo-EM structure at 3.1 Å resolution
Sevvana, M.; Long, F.; Miller, A. S.; Klose, T.; Buda, G.; Sun, L.; Kuhn, R. J.; Rossmann, M. G.
Fernandez, S.; Ostraat, M. L.; Lawrence III, J. A.; Zhang, K.
Local nanoscale strain mapping of a metallic glass during in situ testing
Gammer, C.; Ophus, C.; Pekin, T. C.; Eckert, J.; Minor, A. M.
Xu, H. Lebrette, H.; Yang, T.; Hovmoller, S.; Hogbom, M.; Zou, X.
Hepp, S. E.; Borgo, G. M.; Ticau, S.; Ohkawa, T.; Welch, M. D.
Nanoparticle interactions guided by shape‐dependent hydrophobic forces
Tan, S. F.; Raj, S.; Bisht, G.; Annadata, H. V.; Nijhuis, C. A.; Král, P.; Mirsaidov, U.
Lutz, L.; Dachraoui, W.; Demortière, A.; Johnson, L. R.; Bruce∥, P. G.; Grimaud, A.; Tarascon, J. -M.
Destefani, T. A.; Onaga, G. L.; de Farias, M. A.; Percebom, A. M.; Sabadini, E.
Andrew, R. S.; Austin, B. F.; George, C. R.; Ronald, C. B.; James, M. J.
Solvent-free and biocompatible multiphased organic–inorganic hybrid nanocomposites
da Silva, L. C. E.; Germiniani, L. G. L.; Plivelic, T. S.; Gonçalves, M. C.
S.E.Gilliland III, Tengco, J. M. M.; Yang, Y.; Regalbuto, J. R.; Castano, C. E.; Gupton, B. F.
In situ nanobeam electron diffraction strain mapping of planar slip in stainless steel
Pekin, T. C.; Gammer, C.; Ciston, J.; Ophus, C.; Minor, A. M.
Direct microscopic analysis of individual C60 dimerization events: Kinetics and mechanisms
Okada, S.; Kowashi, S.; Schweighauser, L.; Yamanouchi, K.; Harano, K.; Nakamura, E.
Direct observation of the nanoscale Kirkendall effect during galvanic replacement reactions
Chee, S. W.; Tan, S. F.; Baraissov, Z.; Bosman, M.; Mirsaidov, U.
Atomic structure of sensitive battery materials and interfaces revealed by cryo–electron microscopy
Li, Y.; Li.; Y.; Pei, A.; Yan, K.; Sun, Y.; Wu, C. -L; Joubert, L. -M.; Chin, R.; Koh, A. L.; Yu, Y.; Perrino, J.; Butz, B.; Chu, S.; Cui, Y.
Off-axis electron holography of bacterial cells and magnetic nanoparticles in liquid
Prozorov, T.; Almeida, T. P.; Kovacs, A.; Dunin-Borkowski, R. E.
Breaking cryo-EM resolution barriers to facilitate drug discovery
Merk, A.; Bartesaghi, A.; Banerjee, S.; Falconieri,V.; Rao, P.; Davis, M.; Pragani, R.; Boxer, M.; Earl, L. A.; Milne, J. L. S.; Subramaniam, S.
Han, C. W.; Choksi, T.; Milligan, C.; Majumdar, P.; Manto, M.; Cui, Y.; Sang, X.; Unocic, R. R.; Zemlyanov, D.; Wang, C,; Ribeiro, F. H.; Greeley, J.; Ortalan, V.
Vertical graphene growth on SiO microparticles for stable lithium ion battery anodes
Shi, L.; Pang, C.; Chen, S.; Wang, M.; Wang, K.; Tan, Z.; Gao, P.; Ren, J.; Huang. Y.; Peng, H.; Liu, Z.
Koh, A. L.; Sinclair, R.
Ultrafast electron microscopy integrated with a direct electron detection camera
Lee, Y. M.; Kim, Y. J.; Kim, Y. -J.; Kwon, O. -H.
Optimizing disk registration algorithms for nanobeam electron diffraction strain mapping
Pekin, T. C.; Gammer, C.; Ciston. J.; Minor, A. M.; Ophus, C.
Sinclair, R.; Lee, S. C.; Shi, Y.; Chueh, W. C.
Sato, K.; Yasuda, H.
Oikawa, T.; Okumura, M.; Kimura, T.; Nishiyama, Y.
Gallagher, J. R.; Torian, U.; McCraw, D. M.; Harris, A. K.
Amyloidogenicity and toxicity of the reverse and scrambled variants of amyloid-β 1-42
Vadukul, D. M.; Gbajumo, O.; Marshall, K. E.; Serpell, L. C.
Buha, J.; Manna, L.
Ophus, C.; Ercius, P.; Huijben, M.; Ciston, J.
Gallagher, J. R.; Torian, U.; McCraw, D. M.; Harris, A. K.
Dang, Z.; Shamsi, J.; Palazon, F.; Imran, M.; Akkerman, Q. A.; Park, S.; Bertoni, G.; Prato, M.; Brescia, R.; Manna, L.
Ophus, C.; Rasool, H. I.; Linck, M.; Zettl, A.; Ciston, J.
Distinct nanoscale reaction pathways in a sulfide material for sodium and lithium batteries
Boebinger, M. G.; Xu, M.; Ma, X.; Chen, H.; Unocic, R. R.; McDowell, M. T.
Real-time molecular scale observation of crystal formation
Schreiber, R. E.; Houben, L.; Wolf, S. G.; Leitus, G.; Lang, Z-, L.; Carbó, J. J.; Poblet, J. M.; Neumann, R.
Real-time imaging of self-organization and mechanical competition in carbon nanotube forest growth
Balakrishnan, V.; Bedewy, M.; Meshot, E. R.; Pattinson, S. W.; Polsen, E. S.; Laye, F.; Zakharov, D. N.; Stach, E. A.; Hart, A. J.
Koh, A. L.; Gidcumb, E.; Zhou, O.; Sinclair, R.
Orientation mapping of semicrystalline polymers using scanning electron nanobeam diffraction
Panova, O.; Chen, X. C.; Bustillo, K. C.; Ophus, C.; Bhatt, M. P.; Balsara, N.; Minor, A. M.
Almeida, T. P.; Muxworthy, A. R.; Kovács, A.; Williams, W.; Nagy, L.; Conbhuí, P. O.; Frandsen, C.; Supakulopas, R.; Dunin-Borkowski, R. E.
Analysis of nanoparticle growth in environmental transmission electron microscopy
Mégret, R.; Yoo, S.; Zakharov, D.; Stach, E.
Local and transient nanoscale strain mapping during in situ deformation
Gammer, C.; Kacher, J.; Czarnik, C.; Warren, O. L.; Ciston, J.; Minor, A. M.
Characterization of the nanoscale structure of milk fat
Ramel Jr., P. R. R.; Peyronel, F.; Marangoni, A. G.
Electron-beam induced transformations of layered tin dichalcogenides
Sutter, E.; Huang, Y.; H.-P. Komsa, H. -P.; Ghorbani-Asl, M.; Krasheninnikov, A. V.; Sutter, P.
Practical aspects of diffractive imaging using an atomic-scale coherent electron probe
Chen, Z.; Weyland, M.; Ercius, P.; Ciston, J.; Zheng, C.; Fuhrer, M. S.; D'Alfonso. A. J.; Allen, L. J.; Findlay, S. D.
Islam, E.; Sar, P.
Alavijeh, A. S.; Venkatesan, S. V.; Khorasany, R. M. H.; Kim, W. H. J.; Kjeang, E.
Alibolandi, M.; Alabdollah, F.; Sadeghi, F.; Mohammadi, M.; Abnous, K.; Ramezani, M.; Hadizadeh, F.
The antioxidant protein Oxr1 influences aspects of mitochondrial morphology
Wu, Y.; Davies, K. E.; Oliver, P. L.
Water-induced nanochannel networks in self-assembled block ionomers
Mineart, K. P.; Al-Mohsin, H. A.; Lee, B.; Spontak, R. J.
New form of electron-beam imaging can see elements that are 'invisible' to common methods
Ophus, C.; Ciston, J.; Pierce, J.; Harvey, T. R.; Chess, J.; McMorran, B. J.; Czarnik, C.; Rose, H. H.; Ercius, P.
Fate of silver nanoparticles in wastewater and immunotoxic effects on rainbow trout
Bruneau, A.; Turcotte, P.; Pilote, M.; Gagné, F.; Gagnon, C.
A transmission Kikuchi diffraction study of cementite in a quenched and tempered steel
Saleh, A. A.; Casillas, G.; Pereloma, E. V.; Carpenter, K. R.; Killmore, C. R.; Gazder, A. A.
Radio frequency coplanar ZnO Schottky nanodiodes processed from solution on plastic substrates
Semple, J.; Rossbauer, S.; Burgess, C. H.; Zhao, K.; Jagadamma, L. K.; Amassian, A.; McLachlan, M. A.; Anthopoulos, T. D.
Gating of acoustic transducer channels is shaped by biomechanical filter processes
Hummel, J.; Schöneich, S.; Kössl, M.; Scherberich, J.; Hedwig, B.; Prinz, S.; Nowotny, M.
Strength gradient enhances fatigue resistance of steels
Ma, Z.; Liu, J.; Wang, G.; Wang, H.; Wei, Y.; Gao, H.
Wang, C.; Stanciu, C. E.; Ehrhardt, C. J.; Yadavalli, V. K.
Intact urothelial barrier function in a mouse model of ketamine induced voiding dysfunction
Rajandram, R.; Ong, T. A.; Razack, A. H. A.; MacIver, B.; Zeidel, M.; Yu, W.
Kumar, V. B.; Ezersky, V.; Gedanken, A.; Porat, Z.
Kovács, A.; Pradeep, K. G.; Herzer, G.; Raabe, D.; Dunin-Borkowski, R. E.
Pancreatic β-cell membrane fluidity and toxicity induced by human islet amyloid polypeptide species
Pilkington, E. H.; Gurzov, E. N.; Kakinen, A.; Litwak, S. A.; Stanley, W. J.; Davis, T. P.; Ke, P. C.
Parmenter, C. D. J.; Fay, M. W.; Hartfield, C.; Eltaher, H. M.
Highly mobile ferroelastic domain walls in compositionally graded ferroelectric thin films
Agar, J. C.; Damodaran, A. R.; Okatan, M. B.; Kacher, J.; Gammer, C.; Vasudevan, R. K.; Pandya, S.; Dedon, L. R.; Mangalam, R. V. K.; Velarde, G. A.; Jesse, S.; Balke, N.; Minor, A. M.; Kalinin, S. V.; Martin, L. W.
Performance of a direct detection camera for off-axis electron holography
Chang, S. L. Y.; Dwyer, C.; Barthel, J.; Boothroyd, C. B.; Dunin-Borkowski, R. E.
Hauser, C.; Richter, T.; Homonnay, N.; Eisenschmidt, C.; Qaid, M.; Deniz, H.; Hesse, D.; Sawicki, M.; Ebbinghaus, S. G.; Schmidt, G.
2.3 Å resolution cryo-EM structure of human p97 and mechanism of allosteric inhibition
Banerjee, S.; Bartesaghi, A.; Merk, A.; Rao, P.; Bulfer, S. L.; Yan, Y.; Green, N.; Mroczkowski, B.; Neitz, R. J.; Wipf, P.; Falconieri, V.; Deshaies, R. J.; Milne, J. L.; Huryn, D.; Arkin, M.; Subramaniam, S.
Penicillin G-induced chlamydial stress response in a porcine strain of Chlamydia pecorum
Leonard,C. A.; Dewez, F.; Borel, N.
Zhou, J.; Ghoroghi, S.; Benito-Martin, A.; Wu, H.; Unachukwu, U. J.; Einbond, L. S.; Guariglia, S.; Peinado, H.; Redenti, S.
Torsional deformations in subnanometer MoS interconnecting wires
Koh, A. L.; Wang, S.; Ataca, C.; Grossman, J. C.; Sinclair, R.; Warner, J. H.
Mitochondrial remodeling in chicken induced pluripotent stem like cells
Choi, H. W.; Kim, J. S.; Choi, S.; Hong, Y. J.; Byun, S. J.; Seo, H. G.; Do, J. T.
A mechanism of viral immune evasion revealed by cryo-EM analysis of the TAP transporter
Oldham, M. L., Hite, R. K.; Steffen, A. M.; Damko, E.; Li, Z.; Walz, T.; Chen, J.
Liu, J.; Dupree, J. L.; Gacias, M.; Frawley, R.; Sikder, T.; Naik, P.; Casaccia, P.
The role of mitochondrial membrane spherules in flock house virus replication
Short, J. R.; Speir, J. A.; Gopal, R.; Pankratz, L. M.; Lanman, J.; Schneemann, A.
Shen, J.; Pang, J.; Xu, G.; Xin, X.; Yang, Y.; Luan, X.; Yuan, S.
Eshpari, H.; Tong, P. S.; Corredig, M.
α-Synuclein and huntingtin exon 1 amyloid fibrils bind laterally to the cellular membrane
Monsellier, E.; Bousset, L.; Melki, R.
Harshman, S. W.; Canella, A.; Ciarlariello, P. D.; Agarwal, K.; Branson, O. E.; Rocci, A.; Cordero, H.; Phelps, M. A.; Hade, E. M.; Dubovsky, J. A.; Palumbo, A.; Rosko, A.; Byrd, J. C.; Hofmeister, C. C.; Benson, D. M.; Paulaitis, M. E.; Freitas, M. A.; Pichiorri, F.
In situ study of Fe3Pt–Fe2O3 core–shell nanoparticle formation
Liang, W. I.; Zhang, X.; Zan, Y.; Pan, M.; Czarnik, C.; Bustillo, K.; Xu, J.; Chu, Y. H.; Zheng, H.
Nahirney, P. C.; Reeson, P.; Brown, C. E.
Dynamical features of the plasmodium falciparum ribosome during translation
Sun, M.; Li, W.; Blomqvist, K.; Das, S.; Hashem, Y.; Dvorin, J. D.; Frank, F.
Structure of a yeast spliceosome at 3.6-angstrom resolution
Yan, C.; Hang, J.; Wan, R.; Huang, M.; Wong, C. C. L.; Shi, Y.
An atomic structure of human c-secretase
Bai, X. C.; Yan, C.; Yang, G.; Lu, P.; Ma, D.; Sun, L.; Zhou, R.; Scheres, S. H.; Shi, Y.
In situ structural analysis of golgi intracisternal protein arrays
Engel, B. D.; Schaffer, M.; Albert, S.; Asano, S.; Plitzko, J. M.; Baumeister, W.
Diffraction contrast imaging using virtual apertures
Gammer, C.; Ozdol, V. B.; Liebscher, C. H.; Minor, A. M.
Kitagawa, T.; Kiriyama, K.; Shimizu, Y.
Synthesis of nanostructures in nanowires using sequential catalyst reactions
Panciera, F.; Chou, Y. -C.; Reuter, M. C.; Zakharov, D.; Stach, E. A.; Hofmann, S.; Ross, F. M.
How egg case proteins can protect cuttlefish offspring?
Cornet, V.; Henry, J.; Goux, D.; Duval, E.; Bernay, B.; Le Corguillé, G.; Corre, E.; Zatylny-Gaudin, C.
Strain mapping at nanometer resolution using advanced nano-beam electron diffraction
Ozdol, V. B.; Gammer,C.; Jin, X. G.; Ercius, P.; Ophus, C.; Ciston,J.; Minor, A. M.
Ghosh, T.; Bardhan, M.; Bhattacharya, M.; Satpati, B.
Grant, T.; Grigorieff, N.
Grant, T.; Grigorieff, N.
Electron cryomicroscopy observation of rotational states in a eukaryotic V-ATPase
Zhao, J.; Benlekbir, S.; Rubinstein, J. L.
Structural basis of human γ-secretase assembly
Sun, L.; Zhao, L.; Yang, G.; Yan, C.; Zhou, R.; Zhou, X.; Xie, T.; Zhao, Y.; Wu, S.; Li, X.; Shi, Y.
Structures of the CRISPR-Cmr complex reveal mode of RNA target positioning
Taylor, D. W.; Zhu, Y.; Staals, R. H. J.; Kornfeld, J. E.; Shinkai, A.; van der Oost, J.; Nogales, E.; Doudna, J. A.
Electron cryotomography of vitrified cells with a Volta phase plate
Fukuda, Y.; Laugks, U.; Lučić, V.; Baumeister, W.; Danev, R.
Gedanken, A.; Kumar, V. B.; Porat, Z. I.; Kimmel, G.
Structure of the TRPA1 ion channel suggests regulatory mechanisms
Paulsen, C. E.; Armache, J.; Gao, Y.; Cheng, Y.; Julius, D.
Residual stress and crack initiation in laser clad composite layer with Co-based alloy and WC+NiCr
Lee, C.; Park, H.; Yoo, J.; Lee, C.; Woo, W.C.; Park, S.
Optimising electronholography in the presence of partial coherence and instrument instabilities
Chang, S. L.; Dwyer, C.; Boothroyd, C. B.; Dunin-Borkowski, R. E.
Chen, Y. -T.; Huang, T. -W.; Wang, C. -L.; Hsu, C. -S.
Contractile activity is required for Z-disc sarcomere maturation in vivo
Geach, T. J.; Hirst, E. M. A.; Zimmerman, L. B.
Menegazzo, F.; Signoretto, M.; Marchese, D.; Pinna, F.; Manzoli, M.
Strauss, M.; Filman, D. J.; Belnap, D. M.; Cheng, N.; Noel, R. T.; Hogle, J. M.
Hori, A.; Peddie, C. J.; Collinson, L. M.; Toda, T.;
Cryo-EM structure of influenza virus RNA polymerase complex at 4.3 Å resolution
Chang, S.; Sun, D.; Liang, H.; Wang, J.; Li, J.; Guo, L.; Wang, X.; Guan, C.; Boruah, B. M.; Yuan, L.; Feng, F.; Yang, M.; Wang, L.; Wang, Y.; Wojdyla, J.; Li, L.; Wang, J.; Wang, M.; Cheng, G.; Wang, H. -W.; Liu, Y.
Fibriansah, G.; Tan, J. L.; Smith, S. A.; de Alwis, R.; Ng, T. S.; Kostyuchenko, V. A.; Jadi, R. S.; Kukkaro, P.; de Silva, A. M.; Crowe, J. E.; Lok, S. M.
Conformational changes leading to T7 DNA delivery upon interaction with the bacterial receptor
González-García, V. A.; Pulido-Cid, M.; Garcia-Doval, C.; Bocanegra, R.; van Raaij, M. J.; Martín-Benito, J.; Cuervo, A.; Carrascosa, J. L.
Bruňanská, M.; Bílý, T.; Nebesářová, J.
Ueno, Y.; Mine, S.; Kawasaki, K.
Mechanistic insights into the recycling machine of the SNARE complex
Zhao, M.; Wu, S.; Zhou, Q.; Vivona, S.; Cipriano, D. J.; Cheng, Y.; Brunger, A. T.
Architecture of the RNA polymerase II-Mediator core transcription initiation complex
Plaschka, C.; Lariviere, L.; Wenzeck, L.; Hemann, M.; Tegunov, D.; Petrotchenko, E. V.; Borchers, C. H.; Baumeister, W.; Herzog, F.; Villa, E.; Cramer, P.
Pan, J.; Zhang, L.; Odenwald, M. A.; Shen, L.; Turner, J. R.; Bergelson, J. M.
A look into amyloid formation by transthyretin: aggregation pathway and a novel kinetic model
Faria, T. Q.; de Almeida, Z. L.; Cruz, P. C.; Jesus, C. S. H.; Castanheira, P.; Brito, R. M. M.
Dai, X.; Gong, D.; Xiao, Y.; Wu, T. T.; Sun, R.; Zhou, Z. H.
Alibolandi, M.; Ramezani, M.; Abnous, K.; Sadeghi, F.; Hadizadeh, F.
A molecular census of 26S proteasomes in intact neurons
Asano, S.; Fukuda, Y.; Beck, F.; Aufderheide, A.; Förster, F.; Danev, R.; Baumeister, W.
Strauss, M.; Filman, D. J.; Belnap, D. M.; Cheng, N.; Noel, R. T.; Hogle, J. M.
Visualization of the type III secretion sorting platform of Shigella flexneri
Hu, B.; Morado, D. R.; Margolin, W.; Rohde, J. R.; Arizmendi, O.; Picking, W. L.; Picking, W. D.; Liu, J.
Organization of the mitochondrial translation machinery studied in situ by cryoelectron tomography
Pfeffer, S.; Woellhaf, M. W.; Herrmann, J. M.; Förster, F.
Perrigue, P. M.; Silva, M. E.; Warden, C. D.; Feng, N. L. Reid, M. A.; Mota, D. J.; Joseph, L. P.; Tian, Y. I.; Glackin, C. A.; Gutova, M.; Najbauer, J.; Aboody, K. S.; Barish, M. E.
Native architecture of the Chlamydomonas chloroplast revealed by in situ cryo-electron tomography
Engel, B. D.; Schaffer, M.; Cuellar, L. K.; Villa, E.; Plitzko, J. M.; Baumeister, W.
Rqc2p and the 60S ribosome mediate mRNA-independent elongation of nascent chains
Shen, P. S.; Park, J.; Qin, Y.; Li, X.; Parsawar, K.; Larson, M. H.; Cox, J.; Cheng, Y.; Lambowitz, A. M.; Weissman, J. S.; Brandman, O.; Frost, A.
Pattinson, S. W.; Viswanath, B.; Zakharov, D. N.; Li, J.; Stach, E. A.; Hart, A. J.
Wei-Hau, P.; Yen-Chia, L.; Yat-Pang, C.; Kuo-Shyan, L.; Hsiu-Ni, K.
Lear, P. V.; González-Touceda, D.; Couto, B. P.; Viaño, P.; Guymer, V.; Remzova, E.; Tunn, R.; Chalasani, A.; García-Caballero, T.; Hargreaves, I. P.; Tynan, P. W.; Christian, H. C.; Nogueiras, R.; Parrington, J.; Diéguez, C.
Borges, J.; Buljan, M.; Sancho-Parramon, J.; Bogdanovic-Radovic, I.; Siketic, Z.; Scherer, T.; Kübel, C.; Bernstorff, S.; Cavaleiro, A.; Vaz, F.; Rolo, A. G.
Pageot, J.; Rouzaud, J. -N.; Ahmad, M. A.; Deldicque, D.; Gadiou, R.; Dentzer, J.; Gosmain, L.
Zamurs, L. K.; Idoate, M. A.; Hanssen, E.; Gomez-Ibañez, A.; Pastor, P.; Lamandé, S. R.
Seeing tobacco mosaic virus through direct electron detectors
Fromm, S. A.; Bharat, T. A.; Jakobi, A. J.; Hagen, W. J.; Sachse, C.
Dalui, A.; Thupakula, U.; Khan, A. H.; Ghosh, T.; Satpati, B.; Acharya, S.
Multivalency in the inhibition of oxidative protein folding by arsenic(III) species
Sapra, A.; Ramadan, D.; Thorpe, C.
Anisotropic oxygen ion conduction in melilite intermediate temperature electrolytes
Wei, F.; Gasparyan, H.; Keenan, P. J.; Gutmann, M. J.; Fang, Y.; Baikie, T.; Claridge, J.; Slater, P.; Kloc, C.; White, T.
Novel lift-off technique for transmission electron microscopy imaging of block copolymer films
Roache, F. J. M.; Radjainia, M.; Williams, D. E.; Gerrard, J. A.; Travas-Sejdic, J.; Malmstrӧm, J.
Shang, Z.; Zhou, K.; Xu, C.; Csencsits, R.; Cochran, J. C., Sindelar, C. V.
TORC1 regulators Iml1/GATOR1 and GATOR2 control meiotic entry and oocyte development in Drosophila
Wei, Y.; Reveal, B.; Reich, J.; Laursen, W. J.; Senger, S.; Akbar, T.; Iida-Jones, T.; Cai, W.; Jarnik, M.; Lilly, M. A.
Woodward, C. L.; Cheng, S. N.; Jensen, G. J.
Woodward, C. L.; Cheng, S. N.; Jensen, G. J.
A facile and versatile synthesis of highly luminescent carbon dots-on-glass
Kim, Y.; Namgung, H.; Kim, Y.; Jang, G.; Lee, T. S.
Allosteric communication in the dynein motor domain
Bhabha, G.; Cheng, H.; Zhang, N.; Moeller, A.; Liao, M.; Speir, J. A.; Cheng, Y.; Vale, R. D.
Peng, S.; Wang, K.; Guo, D. S.; Liu, Y.
Organization of capsid-associated tegument components in Kaposi's sarcoma-associated herpesvirus
Dai, X.; Gong, D.; Wu, T. T.; Sun, R.; Zhou, Z. H.
Tsiapa, I.; Efthimiadou, E. K.; Fragogeorgi, E.; Loudos, G.; Varvarigou, A. D.; Bouziotis, P.; Kordas, G. C.; Mihailidis, D.; Nikiforidis, G. C.; Xanthopoulos, S.; Psimadas, D.; Paravatou-Petsotas, M.; Palamaris, L.; Hazle, J. D.; Kagadis, G. C.
Near-atomic resolution reconstructions using a mid-range electron microscope operated at 200 kV
Campbell, M. G.; Kearney, B. M.; Cheng, A.; Potter, C. S.; Potter, C. S.; Carragher, B.; Veesler, D.
Bharat, L. K.; Rao, B. V.; Yu, J. S.
LDH and TiO2/LDH-type nanocomposite systems: a systematic study on structural characteristics
Seftel, E. M.; Niarchos, M.; Vordos, N.; Nolan, J. W.; M. Mertens, M.; Mitropoulos, A. Ch.; Vansant, E. F.; Cool, P.
Nakamura, T.; Hiyoshi, N.; Hayashi, H.; Ebina, T.
Poddighe, S.; De Rose, F.; Marotta, R.; Ruffilli, R.; Fanti, M.; Secci, P. P.; Mostallino, M. C.; Setzu, M. D.; Zuncheddu, M. A.; Collu, I.; Solla, P.; Marrosu, F.; Kasture, S.; Acquas, E.; Liscia, A.
Singh, R.; Dong, H.; Liu, D.; Zhao, L.; Marts, A. R.; Farquhar, E.; Tierney, D. L.; Almquist, C. B.; Briggs, B. R.
Hakeem, A. A.; Zhao, Z.; Kapteijn, F.; Makkee, M.
Lehofer, B.; Bloder, F.; Jain, P. P.; Marsh, L. M.; Leitinger, G.; Olschewski, H.; Leber, R.; Olschewski, A.; Prassl, R.
Ramamurthy V1, Jolicoeur C2, Koutroumbas D.; Mühlhans, J.; Le, Y. Z.; Hauswirth, W. W.; Giessl, A.; Cayouette, M.
Subnanometre-resolution electron cryomicroscopy structure of a heterodimeric ABC exporter
Kim, J.; Wu, S.; Tomasiak, T. M.; Mergel, C.; Winter, M. B.; Stiller, S. B.; Robles-Colmanares, Y.; Stroud, R. M.; Tampé, R.; Craik, C. S.; Cheng, Y.
Zhao, P.; Hao, J.
Visualization of ATP synthase dimers in mitochondria by electron cryo-tomography
Davies, K. M.; Daum, B.; Gold, V. A.; Mühleip, A. W.; Brandt, T.; Blum, T. B.; Mills, D. J.; Kühlbrandt, W.
Cheng, C.; Gu, J.; Su, J.; Ding, W.; Yin, J.; Liang, W.; Yu, X.; Ma, J.; Wang, P. G.; Xiao, Z.; Liu, Z. -J.
Volkmann, N.; Martásek, P.; Roman, L. J.; Xu, X. P.; Page, C.; Swift, M.; Hanein, D.; Masters, B. S.
Molecular imprinting as a signal-activation mechanism of the viral RNA sensor RIG-I
Wu, B.; Peisley, A.; Tetrault, D.; Li, Z.; Egelman, E. H.; Magor, K. E.; Walz, T.; Penczek, P. A.;Hur, S.
Mineralogy and petrology of comet 81P/wild 2 nucleus samples
Zolensky, M. E.; Zega, T. J.; Yano, H.; Wirick, S.; Westphal, A. J.; Weisberg, M. K.; Weber, I.; Warren, J. L.; Velbel, M. A.; Tsuchiyama, A.; Tsou, P.; Toppani, A.; Tomioka, N.; Tomeoka, K.; Teslich, K.; Taheri, M.; Susini, J.; Stroud, R.; Stephan, T.; Stadermann, F. J.; Snead, C. J.; Simon, S. B.; Simionovici, A.; See, T. H.; Robert, F.; Rietmeijer, F. J. M.; Rao, W.; Perronnet, M. C.; Papanastassiou, D. A.; Okudaira, K.; Ohsumi, K.; Ohnishi, I.; Nakamura-Messenger, K.; Nakamura, T.; Mostefaoui, S.; Mikouchi, T.; Meibom, A.; Matrajt, G.; Marcus, M. A.; Leroux, H.; Lemelle, L.; Le, L.; Lanzirotti, A.; Langenhorst, F.; Krot, A. N.; Keller, L. P.; Kearsley, A. T.; Joswiak, D.; Jacob, D.; Ishii, H.; Harvey, R.; Hagiya, K.; Grossman, L.; Grossman, J. N.; Graham, G. A.; Gounelle, M.; Gillet, P.; Genge, M. J.; Flynn, G.; Ferroir, T.; Fallon, S.; Ebel, D. S.; Dai, Z. D.; Cordier, P.; Clark, B.; Chi, M.; Butterworth, A. L.; Brownlee, D. E.; Bridges, J. C.; Brennan, S.; Brearley, A.; Bradley, J. P.; Bleuet, P.; Bland, P. A.; Bastien, R.
Facet development during platinum nanocube growth
Liao, H. G.; Zherebetskyy, D.; Xin, H.; Czarnik, C.; Ercius, P.; Elmlund, H.; Pan, M.; Wang, L. W.; Zheng, H.
Structure of β-galactosidase at 3.2-Å resolution obtained by cryo-electron microscopy
Bartesaghi, A.; Matthies, D.; Banerjee, S.; Merk, A.; Subramaniam, S.
Single particle electron cryo-microscopy of a mammalian ion channel
Liao, M.; Cao, E.; Julius, D.; Cheng, Y.
Influence of a single grain boundary on domain wall motion in ferroelectrics
Marincel, D. M.; Zhang, H.; Kumar, A.; Jesse, S.; Kalinin, S. V.; Rainforth, W. M.; Reaney, I. M.; Randall, C. A.; Trolier-McKinstry, S.
Kitta, M.; Akita, T.; Tanaka, S.; Kohyama, M.
M-free: Scoring the reference bias in sub-tomogram averaging and template matching
Yu, Z.; Frangakis, A. S.
Shami, G.; Cheng, D.; Henriquez, J.; Braet, F.
Structural and morphological investigations of β-cyclodextrin-coated silver nanoparticles
Andrade, P. F.; de Faria, A. F.; da Silva, D. S.; Bonacin, J. A.; Gonçalves, M. C.
Zhu, X. Q.; Feng, Q.; Liu , D. Z.; Nie, A. M.; Liu, J. B.; Zhang, X. B.; Geng, L. M.
Mu, X.; Sigle, W.; Bach, A.; Fischer, D.; Jansen, M.; van Aken, P. A.
Akita, T.; Tabuchi, M.; Nabeshima, Y.; Tatsumi, K.; Kohyama, M.
Visualizing active membrane protein complexes by electron cryotomography
Gold, V. A. M.; Ieva, R.; Walter, A.; Pfanner, N.; van der Laan, M.; Kühlbrandt, W.
Abnormalities associated with grain growth in solid solution Cu(Ni) thin films
Brons, J. G.; Thompson, G. B.
CTER-rapid estimation of CTF parameters with error assessment
Penczek, P. A.; Fang, J.; Li, X.; Cheng, Y.; Loerke, J.; Spahn, C. M. T.
Investigation of the microcrack evolution in a Ti-based bulk metallic glass matrix composite
Wang, Y.; Guo, Z.; Ma, R.; Hao, G.; Zhang, Y.; Lin, J.; Sui, M.
Cho, H. J.; Hyun, J. K.; Kim, J. G.; Jeong, H. S.; Park, H. N.; You, D. J.; Jung, H. S.
Structure of bacterial cytoplasmic chemoreceptor arrays and implications for chemotactic signaling
Briegel, A.; Ladinsky, M.S.; Oikonomou, C.; Jones, C. W.; Harris, M. J.; Fowler, D. J.; Chang, Y. W.; Thompson, L. K.; Armitage, J. P.; Jensen, G. J.
In-situ TEM study of stability of TaRhx diffusion barriers using a novel sample preparation method
Dalili, N.; Li, P.; Kupsta, M.; Liu, Q.; Ivey, D. G.
New insights into bacterial chemoreceptor array structure and assembly from electron cryotomography
Briegel, A.; Wong , M. L.; Hodges, H. L.; Oikonomou, C. M.; Piasta, K. N.; Harris, M. J.; Fowler, D. J.; Thompson L. K.; Falke, J. J.; Kiessling, L. L; Jensen, G. J.
Gil, S.; Cuenca, N.; Romero, A.; Valverde, J. L.; Sánchez-Silva, L.
Structural dynamics of viral nanomachines
Kam, J.; Demmert, A. C.; Tanner, J. R.; McDonald, S. M.; Kelly, D. F.
Shahmoradian, S. H.; Galiano, M. R.; Chengbiao Wu, C.; Chen, S.; Rasband, M. N.; Mobley, W. C.; Chiu, W.
Reduction and immobilization of hexavalent chromium by microbially reduced Fe-bearing clay materials
Bishop, M. E.; Glasser, P.; Dong, H.; Arey, B. W.; Kovarik, L.
Influence of turbostratic carbon nanostructures on electrical conductivity in shales
Walters, C. C.; Kliewer, C. E.; Awwiller, D. N.; Rudnicki, M. D.;Passey, Q. R.; Lin, M. W.
Architecture and host interface of environmental chlamydiae revealed by electron cryotomography
Pilhofer, M.; Aistleitner, K.; Ladinsky, M. S.; König, L.; Horn, M.; Jensen, G. J.
Watanabe, S.; Kinoshita, M.; Hosokawa, T.; Morigaki, K.; Nakura, K.
Structural basis for the prion-like MAVS filaments in antiviral innate immunity
Xu, H.; He, X.; Zheng, H.; Huang, L. J.; Hou, F.; Yu, Z.; de la Cruz, M. J.; Borkowski, B.; Zhang, X.; Chen, Z. J.; Jiang, Q. X.
Balasubramaniam, S.; Kayandan, S.; Lin, Y. N.; Kelly, D. F.; House, M. J.; Woodward, R. C.; St. Pierre, T. G.; Riffle, J. S.; Davis, R. M.
Mechrez, G.; Krepker, M. A.; Harel, Y.; Lellouchec, J. P.; Segal, E.
Practical workflow for cryo focused-ion-beam milling of tissues and cells for cryo-TEM tomography
Hsieh, C.; Schmelzer, T.; Kishchenko, G.; Wagenknecht, T.; Marko, M.
On resolution in electron tomography of beam sensitive materials
Chen, D.; Friedrich, H.; de With, G.
A quantitative recipe for engineering protein polymer nanoparticles
Janib, S. M.; Pastuszka, M. F.; Aluri, S.; Folchman-Wagner, Z.; Hsueh, P. Y.; Shi, P.; Lin, Y. A.; Cuib H.; MacKay, J. A.
Carretero-Genevrier, A.; Oro-Sole, J.; Gazquez, J.; Magén, C.; Miranda, L.; Puig, T.; Obradors, X.; Ferain, E.; Sanchez, C.; Rodriguez-Carvajal, J.; Mestres, N.
Cryo-fixation by self-pressurized rapid freezing
Grabenbauer, M.; Han, H. M.; Huebinger, J.
Seeing and measuring in colours: Electron microscopy and spectroscopies applied to nano-optics
Kociak, M,; Stéphan, O.; Gloter, A.; Zagonel, L. F.; Tizei, L. H. G.; Tencé, M.; March, K.; Blazit, J. D.; Mahfoud, Z.; Losquin, A.; Meuret, S.; Colliex, C.
Gai, P. L.; Lari, L.; Ward, M. R.; Boyes, E. D.
Size-controlled self-assembly of superparamagnetic polymersomes
Hickey, R. J.; Koski, J.; Meng, X.; Riggleman, R. A.; Zhang, P.; Park, S. J.
Cryo-EM structure of a fully glycosylated soluble cleaved HIV-1 envelope trimer
Lyumkis, D.; Julien, J. P.; de Val, N.; Cupo, A.; Potter, C. S.; Klasse, P. J.; Burton, D. R.; Sanders, R. W.; Moore, J. P.; Carragher, B.; Wilson, I. A.; Ward, A. B.
TRPV1 structures in distinct confirmations reveal activation mechanisms
Cao, E.; Liao, M.; Cheng, Y.; Julius, D.
Cryo-SiN – An alternative substrate to visualize active viral assemblies
Tanner, J. R.; Demmert, A. C.; Dukes, M. J.; Melanson, L. A.; McDonald, S. M.; Kelly, D. F.
In-situ TEM investigation of fracture process in an Al–Cu–Mg alloy
Feng, Z. Q.; Yang, Y. Q.; Chena, Y. X.; Huang, B.; Fu, M. S.; Li, M. H.; Ru, J. G.
Quantitative characterization of electron detectors for transmission electron microscopy
Ruskin, R. S.; Yu, Z.; Grigorieff, N.
Maximizing the potential of electron cryomicroscopy data collected using direct detectors
Veesler, D.; Campbell, M. G.; Cheng, A.; Fu, C.; Murez, Z.; Johnson, J. E.; Potter, C. S.; Carragher, B.
Understanding and controlling low-temperature aging of nanocrystalline materials
Battaile, C. C.; Boyce, B. L.; Brons, J. G.; Foiles, S. M.; Hattar, K. A.; Holm, E. A; Padilla, H. A.; Sharon, J. A.; Thompson, G. B.
Functionalized Au/Ag nanocages as a novel fluorescence and SERS dual probe for sensing
Zoppi, A.; Trigari, S.; Giorgetti, E.; Muniz-Miranda, M.; Alloisio, M.; Demartini, A.; Dellepiane, G.; Thea, S.; Dobrikov, G.; Timtcheva, I.
Fe and Mn oxidation states by TEM-EELS in fine-particle emissions from a Fe-Mn alloy making plant.
Marris, H.; Deboudt, K.; Flament, P.; Grobéty, B.; Gieré, R.
Electrostatic control of structure in self-assembled membranes
Bitton, R.; Chow, L. W.; Zha, R. H.; Velichko, Y. S.; Pashuck, E. T.; Stupp, S. I.
Polyphosphate storage during sporulation in the gram-negative bacterium acetonema iongum
Tocheva, E. I.; Dekas, A. E.; McGlynn, S. E.; Morris, D.; Orphan, V. J.; Jensen, G. J.
Briegel, A.; Ames, P.; Gumbart, J. C.; Oikonomou, C. M.; Parkinson, J. S.; Jensen, G. J.
Kitta, M.; Akita, T.; Tanaka, S.; Kohyama, M.
Influence of electron dose on electron counting images recorded with the K2 camera
Li, X.; Zheng, S. Q.; Egami, K.; Agard, D. A.; Cheng, Y.
Microstructural analysis of dehydrogenation products of the Ca(BH4)2–MgH2 composite
Kim, J. M.; Kim, Y.; Shim, J. H.; Lee, Y. S.; Suh, J. Y.; Ahn, J. P.; Kim, G. H.; Cho, Y. W.
Häussler, D.; Houben, L.; Essig, S.; Kurttepeli, M.; Dimroth, F.; Dunin-Borkowski, R. E.
Peptidoglycan transformations during Bacillus subtilis sporulation
Tocheva, E. I.; López-Garrido, J.; Hughes, H. V.; Fredlund ,J.; Kuru, E.; Vannieuwenhze, M. S.; Brun, Y. V.; Pogliano, K.; Jensen. G. J.
Vargas, G. E.; Haro Durand, L. A.; Cadena, V.; Romero, M.; Mesones, R. V.; Mačković, M.; Spallek, S.; Spiecker, E.; Boccaccini, A. R.; Gorustovich, A. A.
Kai, X.; Zhiqiang Li, Z.; Genlian Fan, G.; Guo, Q.; Tan, Z.; Zhang, W.; Su, Y.; Weijie Lu, W.; Moon, W. J.; Zhang, D.
Sánchez-Santolino, G.; Tornos, J.; Bruno, F. Y.; Cuellar, F. A.; Leon, C.; Santamaría, J.; Pennycook, S. J.; Varela, M.
Klimenkov M.; Möslang A.; Materna-Morris, E.
Construction and organization of a BSL-3 cryo-electron microscopy laboratory at UTMB
Sherman, M. B.; Trujillo, J.; Leahy, I.; Razmus, D.; DeHate, R.; Lorcheim, P.; Czarneskif, M. A.; Zimmerman, D.; Newton, J. T. M.; Haddow, A. D.; Weaver, S. C.
Elucidating elementary processes at Cu/ZnO interfaces: A microscopical approach
Zychma, A.; Wansing, R.; Schott, V.; Köhler, U.; Wöll, C.; Muhler, M.; Birkner, A.
Gandman, M.; Kauffmann, Y.; Koch, C. T.; Kaplan, W. D.
Nishimoto, K.; Sato, T.; Tamura, R.
Plastic deformation of single crystals of Pt3Al with the L12 structure
Okamoto, N. L.; Hasegawa, Y.; Hashimoto, W.; Inui, H.
Wang, M. S.; Hanson, J. P.; Gradečak, S.; Demkowicz, M. J.
Ampelli, C.; Passalacqua, R.; Genovese, C.; Perathoner, S.; Centi, G.; Montini, T.; Gomba, V.; Jaen, J. J. D.; Fornasiero, P.
Thermally robust anion-chain order in oxynitride perovskites
Clark, L.; Oró-Solé, J.; Knight, K. S.; Fuertes, A.; Attfield, J. P.
Gold catalyzed nickel disilicide formation: A new solid–liquid-solid phase growth mechanism
Tang, W.; Picraux, S. T.; Huang, J. H.; Liu, X.; Tu, K. N.; Dayeh, S. A.
Spin transition of Fe2+ in ringwoodite (Mg,Fe)2SiO4 at high pressures
Lyubutin, I. S.; Lin, J. F.; Gavriliuk, A. G.; Mironovich, A. A.; Ivanova, A. G; Roddatis, V. V.; Vasiliev, A. L.
Huang, J.; Hastings, C. L.; Duffy, G. P.; Kelly, H. M.; Raeburn, J.; Adams, D. J.; Heise, A.
Vad, T.; Wulfhorst, J.; Pan, T. T.; Steinmann, W.; Dabringhaus, S.; Beckers, M.; Seide, G.; Gries, T.; Sager, W. F. C.; Heidelmann, M.; Weirich, T. E.
Compression deformation of single-crystal Pt3Al with the L12 structure
Hasegawa, Y.; Okamoto, N. L.; Inui, H.
Evolution of order in amorphous-to-crystalline phase transformation of MgF2
Mu, X.; Neelamraju, S.; Sigle, W.; Koch, C. T.; Totò, N.; Schön, J. C.; Bach, A.; Fischer, D.; Jansen, M.; van Aken, P. A.
Single particle cryo-electron microscopy and 3-D reconstruction of viruses
Guo, F.; Jiang, W.
Seeing the atoms more clearly: STEM imaging from the Crewe era to today
Pennycook, S. J.
Aberration-corrected and energy filtered precession electron diffraction
Eggeman, A. S.; Barnard, J. S.; Midgley, P. A.
Nolin, F.; Ploton, D.; Wortham, L.; Tchelidze, P.; Balossier, G.; Banchet, V.; Bobichon, H.; Lalun, N.; Terryn, C.; Michela, J.
Tracking lithium transport and electrochemical reactions in nanoparticles
Wang, F.; Yu, H. C.; Chen, M. H.; Wu, L.; Pereira, N.; Thornton, K.; Van der Ven, A.; Zhu, Y.; Amatucci, G. G.; Graetz, J.
Strain measurement in semiconductor heterostructures by scanning transmission electron microscopy
Müller, K.; Rosenauer, A.; Schowalter, M.; Zweck, J.; Fritz, R.; Volz, K.
Collins, S. E.; Delgado, J. J.; Mira, C.; Calvino, J. J.; Bernal, S. B.; Chiavassa, D. L.; Baltanás, M. A.; Bonivardi, A. L.
In situ TEM study on elastic interaction between a prismatic loop and a gliding dislocation
Matsukawa, Y.; Liu, G. S.
Interactions of gold nanoparticles with the interior of hollow graphitized carbon nanofibers
La Torre, A.; Fay, M. W.; Rance, G. A.; Gimenez-Lopez, M. d. C.; Solomonsz, W. A.; Brown, P. D.; Khlobystov, A. N.
High-resolution in situ and ex situ TEM studies on graphene formation and growth on Pt nanoparticles
Penga, Z.; Somodia, F.; Helveg, S.; Kisielowski, C.; Specht, P.; Bell, A. T.
STEM characterization for lithium-ion battery cathode materials
Huang, R.; Ikuhara, Y.
Subparticle ultrafast spectrum imaging in 4D electron microscopy
Yurtsever, A.; van der Veen, R. M.; Zewail, A. H.
Schulenburg, H.; Roth, C.; Scheiba, F.
Cosandeya, F.; Suc, D.; Sinaa, M.; Pereiraa, N.; Amatuccia, G. G.
Advanced synthesis of materials for intermediate-temperature solid oxide fuel cells
Shao, Z.
Anisotropic nanocrystal dissolution observation by in situ transmission electron microscopy
Cordeiro, M. A. L.; Crozier, P. A.; Leite, E. R.
Haruta, M.; Kurashima, K.; Nagai, T.; Komatsu, H.; Shimakawa, Y.; Kurata, H.; Kimoto, K.
Majidi, H.; Winkler, C. R.; Taheri, M. L.; Baxter, J. B.
Analysis of few-nm sized metal nanoparticles on carbon nanostructures
Fay, M. W.; La Torre, A.; Khlobystov, A. N.; Brown, P. D.
Ga ordering and electrical conductivity in nanotwin and superlattice-structured Ga-doped ZnO
Yoon, S. W.; Seo, J. H.; Seong, T. Y.; Yu, T. H.; You, Y. H.; Lee, K. B.; Kwon, H.; Ahn, J. P.
Deformation-mediated phase transformation in gold nano-junction
Nie, A.; Wang, H.
Nemcsics, Á.; Heyn, Ch.; Tóth, L.; Dobos, L.; Stemmann, A.; Hansen, W.
Electronic structure of supelattice and twin in Ga doped ZnO measured by monochromated EELS
Chang, H.; Yoon, S.; Seong, T.; Yu, T.; Yuo, Y.; Ahn, J.
Tan, H.; Turner, S.; Yücelen, E.; Verbeeck, J.; Van Tendeloo, G.
Lari, L.; Walther, T.; Gass, M.H.; Geelhaar, L.;Chèze, C.; Riechert, H.; Bullough, T. J.; Chalker, P. R.
Mn-SBA15 catalysts prepared by impregnation: Influence of the manganese precursor
Pérez, H.; Navarro, P.; Delgado, J. J.; Montes, M.
Tuneable mechanical properties in low molecular weight gels
Chen, L.; Raeburn, J.; Sutton, S.; Spiller, D. G.; Williams, J.; Sharp, J. S.; Griffiths, P. C.; Heenan, R. K.; King, S. M.; Paul, A.; Furzelan, S.; Derek Atkins, D.; Adams, D. J.
Mimivirus shows dramatic genome reduction after intraamoebal culture
Boyer, M.; Azza, S.; Barrassi, L.; Klose, T.; Campocasso, A.; Pagnier, I.; Fournous, G.; Borg, A.; Robert, C.; Zhang, X.; Desnues, C.; Henrissat, B.; Rossmann, M. G.; La Scola, B.; Raoulta, D.
EELS characterization of radiolytic products in frozen samples
Aronova, M. A.; Sousa, A. A.; Leapman, R. D.
Janbroers, S.: Crozierc, P. A.; Zandbergen, H. W.; Kooyman, P. J.
Crozier, P. A.; Chenna, S.
Magnetocrystalline anisotropy and uniaxiality of MnAs/GaAs(100) films
Wikberg, J. M.; Knut, R.; Bhandary, S.; di Marco, I.; Ottosson, M.; Sadowski, J.; Sanyal, B.; Palmgren, P.; Tai, C. W. ; Eriksson, O.; Karis, O.; Svedlindh, P.
Structural evolution of magnesium difluoride: from an amorphous deposit to a new polymorph
Bach, A.; Fischer, D.; Mu, X.; Sigle, W.; van Aken, P. A.; Jansen, M.
Native ultrastructure of the red cell cytoskeleton by cryo-electron tomography
Nans, A.; Mohandas, N.; Stokes, D. L.
Schamm-Chardon, S.; Coulon, P.E.; Lamagna, L.; Wiemer, C.; Baldovino, S.; Fanciulli, M.
Vergnat, C.; Landais, V.; Legrand, J. F.; Brinkmann, M.
Synthesis and modelling of gold nanostars with tunable morphology and extinction spectrum
Trigari, S.; Rindi, A.; Margheri, G.; Sottini, S.; Dellepiane, G.; Giorgetti, E.
Mapping titanium and tin oxide phases using EELS: An application of independent component analysis
de la Peña, F.; Berger, M. H.; Hochepied, J. F.; Dynys, F.; Stephan, O.; Walls, M.
Lee, H. S.; Lee, Y. S.; Suh, J. Y.; Kim, M.; Yu, J. S.; Cho, Y. W.
Inceesungvorn, B.; López-Castro, J.; Calvino, J. J.; Bernal, S.; Meunier, F. C.; Hardacre, C.; Griffin, K.; Delgado, J. J.
Hydrogelation of self-assembling RGD-based peptides
Cheng, G.; Castelletto, V.; Jones, R. R.; Connona, C. J.; Hamley, I. W.
Single crystalline La0.7Sr0.3MnO3 molecular sieve nanowires with high temperature ferromagnetism
Carretero-Genevrier, A.; Gázquez, J.; Idrobo, J. C.; Oró, J.; Arbiol, J.; Varela, M.; Ferain, E.; Rodríguez-Carvajal, J.; Puig, T.; Mestres, N.; Obradors, X.
Cryo-electron tomography of microtubules assembled in vitro from purified components
Coquelle, F. M.; Blestel, S.; Heichette, C.; Arnal, I.; Kervrann, C.; Chrétien, D.
Real-time observation of impurity diffusion in silicon nanowires
Holmberg, V. C.; Collier, K. A.; Korgel, B. A.
Low-temperature structural stability of Cd6 M (M = Ho, Er, Tm and Lu) cubic crystalline approximants
Nishimoto, K.; Sato, T.; Muraki, M.; Tamura, R.
Block copolymer self-assembly-directed single-crystal homo- and heteroepitaxial nanostructures
Arora, H.; Du, P.; Tan, K. W.; Hyun, J. K.; Grazul, J.; Xin, H. L.; Muller, D. A.; Thompson, M. O.; Wiesner, U.
D-STEM: A parallel electron diffraction technique applied to nanomaterials
Ganesh, K. J.; Kawasaki, M.; Zhou, J. P.; Ferreira, P. J.
Mishima, T. D.; Edirisooriya, M.; Santos, M. B.
Gentle STEM: ADF imaging and EELS at low primary energies
Krivanek, O. L.; Dellby, N.; Murfitt, M. F.; Chisholm, M. F.; Pennycook, T. J.; Suenaga, K.; Nicolosi, V.
Low-temperature transmission electron microscopy study of superconducting Nb3Sn
Schierning, G.; Theissmann, R.; Acet, M.; Hoelzel, M.; Gruendmayer, J.; Zweck, J.
van Schooneveld, M. M.; Gloter, A.; Stephan, O.; Zagonel, L. F.; Koole, R.; Meijerink, A.; Mulder W. J. M.; de Groot, F. M. F.
Mishima, T. D.; Santos, M. B.
Kim, H. S.; Myung, Y.; Cho, Y. J.; Jang, D. M.; Jung, C. S.; Park, J.
Xu, H.; Das, A. K.; Horie, M.; Shaik, M. S.; Smith, A. M.; Luo, Y.; Lu, X.; Collins, R.; Liem, S. Y.; Song, A.; Popelier, P. L. A.; Turner, M. L.; Xiao, P.; Kinloch, I. A.; Ulijn, R. V.
Electronic tuning of two metals and colossal magnetoresistances in EuWO1+xN2−x perovskites
Yang, M.; Oró-Solé, J.; Kusmartseva, A.; Fuertes, A.; Attfield, J. P.
Wu, C. T.; Chu, M. W.; Chen, L. C.; Chen, K. H.; Chen, C. W.; Chen, C. H.
Orientation dependence of shock-induced twinning and substructures in a copper bicrystal
Cao, F.; Beyerlein, I. J.; Addessio, F. L.; Sencer, B. H.; Trujillo, C. P.; Cerreta, E. K.; Gray III, G. T.
Insights into the nature of iron-based Fischer-Tropsch catalysts from quasi in-situ TEM-EELS and XRD
Janbroers, S.; Louwen, J. N.; Zandbergen, H. W.; Kooyman, P. J.
Servanton, G.; Pantel, R.
Tanaka, K.; Miwa, T.; Sasaki, K.; Kuroda, K.
Bernier, N.; Brosset, C.; Bocquet, F.; Tsitrone, E.; Saikaly, W.; Khodja, H.; Alimov, V. Kh.; Gunn, J. P.
Four-dimensional STEM-EELS: Enabling nano-scale chemical tomography
Jarausch, K.; Thomas, P.; Leonard, D. N.; Twesten, R.; Booth, C. R.
Yakovlev, S.; Libera, M.
Feldhoff, A.; Arnold, M.; Martynczuk, J.; Gesing, Th. M.; Wang, H.
TEM studies of Nb2O5 catalyst in ball-milled MgH2 for hydrogen storage
Porcu, M.; Petford-Long, A. K.; Sykes, J. M.
Optical properties and bandgaps from low loss EELS: Pitfalls and solutions
Stöger-Pollach, M.
Yamada, T.; Maigne, A.; Yudasaka, M.; Mizuno, K.; Futaba, D. N.; Yumura, M.; Iijima, S.; Hata, K.
Complete composition tunability of InGaN nanowires using a combinatorial approach
Kuykendall, T.; Ulrich, P.; Aloni, S.; Yang, P.
Imaging of single organic molecules in motion
Koshino, M.; Tanaka, T.; Solin, N.; Suenaga, K.; Isobe, H.; Nakamura, E.
High-angular-resolution electron energy loss spectroscopy of hexagonal boron nitride
Arenal, R.
Heterogeneity of a vulcanized rubber by the formation of ZnS clusters
Dohi, H.; Horiuchi, S.
Local refinement: An attempt to correct for shrinkage and distortion in electron tomography
Cantele, F.; Zampighi, L.; Radermacher, M.; Zampighi, G.; Lanzavecchia, S.
Blackford, M. G.; Hanna, J. V.; Pike, K. J.; Vance, E. R.; Perera, D. S.
Three-dimensional architecture of virus-packed tubule
Katayama, S.; Wei, T.; Omura, T.; Takagi, J.; Iwasaki, K.
Fe speciation in geopolymers with Si/Al molar ratio of ∼2
Perera, D. S.; Cashion, J. D.; Blackford, M. G.; Zhang, Z.; Vance, E. R.
Novel synthesis of Al2O3 nano-particles by flame spray pyrolysis
Tok, A. I. Y.; Boey, F. Y. C.; Zhao, X. L.
Structure and phase transitions in Ca2CoSi2O7-Ca2ZnSi2O7 solid-solution crystals
Jia, Z. H.; Schaper, A. K.; Massa, W.; Treutmann W.; Rager, H.
Horiuchi, S.; Dohi, H.
Jinschek, J. R.; Erni, R.; Gardner, N. F.; Kim, A. Y.; Kisielowski, C.
Yaicle, C.; Fauth, F.; Martin, C.; Retoux, R.; Jirak, Z.; Hervieu, M.; Raveau, B.; Maignan, A.
Nanoscale analysis of polymer interfaces by energy-filtering transmission electron microscopy
Horiuchi, S.; Yin, D.; Ougizawa, T.
TEM analysis of Gd5(SixGel−x)4, where x=1/2
Meyers, J. S.; Chumbley, S.; Laabs, F.; Pecharsky, A. O.
Nanoscale EELS analysis of dielectric function and bandgap properties in GaN and related materials
Borckt, G.; Lakner, H.
Measuring the thickness of aluminium alloy thin foils using electron energy loss spectroscopy
Bardal, A.; Lie, K.
Longo, D. M.; Howe, J. M.; Johnson, W. C.
Longo, D. M.; Howe, J. M.; Johnson, W. C.
Nyquist frequency
Dose fractionation and motion correction
Improving DQE with counting and super-resolution